Developmental Origins for Neuropsychiatric Illness
In the last two decades, neuropsychiatric investigation focusing on children and adolescents has begun to offer new paradigms for understanding brain-and-behavior relationships. The medical model archetype, long accepted as fundamental for neuropsychiatric underpinning of disease in adults, is becoming more well developed in pediatric psychiatry. Pediatric imaging studies, although challenged by potential movement artifact and necessary avoidance of radiation exposure, have begun to delineate developmental and structural variability in children.9 Furthermore, categorical criteria established for psychiatric diagnoses in adults have become more flexibly applied such that they may be relevant to younger age-groups.10 Identifying symptoms that serve as precursors to illness seen in adults may more efficiently advance understanding of the natural history of disease. Ultimately, it may be intuitive to hypothesize that neuropsychiatric illness identified in adults has anatomic or functional antecedents early in life. In order to fully appreciate the complexities of brain–behavior relationships, it is imperative to understand the developmental stages of the cognitive and emotional pathways in the brain. We present a synopsis of the major pre- and postnatal developmental stages that must occur for normal higher cortical function; these are followed by examples of proposed development abnormalities that lead to pediatric and adult neuropsychiatric disease.
Developmental Stages
Embryo (Conception to 8 Weeks)
Before the formation of the nervous system, there are three main cell layers, the endoderm (forms gastrointestinal tract, lungs, and liver), the mesoderm (forms muscles, connective tissue, and the vascular system) and ectoderm (forms the entire nervous system). During postconception Weeks (PCW) 2–3, the ectoderm forms the neural plate, which then develops the neural groove, bounded bilaterally by neural folds.1,11,12 By PCW 4, the neural tube starts to close. Fusion of the neural folds begins at the cervical level of the future spinal cord and expands rapidly in both rostral and caudal directions.1 Even before the neural tube has finished closing, three local enlargements (primary vesicles or divisions) begin to appear (Figure 1). These give rise to the five secondary divisions which will form the brain (Figure 1).1 Predecessor cells, a very early population of neurons, arrive under the pial surface about this time to begin formation of the preplate (primordial plexiform layer).13 By PCW 5, neuronal precursor cells proliferating in the ventricular zone (germinal matrix) lining the ventricles begin to generate cells that will differentiate into neurons or glia.12,13 They also form a second area of proliferating cells, the subventricular zone. By PCW 8, neuronal migration toward the pial surface has begun. The cortical plate is formed by accumulation of neurons within the preplate. The first temporary synapses are present in marginal zone (above the cortical plate) and presubplate/subplate (below the cortical plate).7,8,12,13 It is likely that these are formed by early afferents from brainstem and basal forebrain.7,8
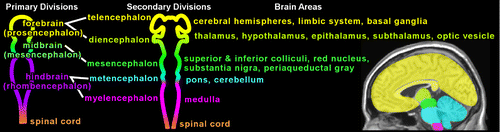
FIGURE 1. The neural tube (illustrated in schematic form) expands locally to form the three primary divisions or vesicles (left).1 These, in turn, form the five secondary divisions (middle). The forebrain vesicle (prosencephalon [yellow]) subdivides into telencephalon (yellow) and diencephalon (light green). The hindbrain vesicle (rhombencephalon [purple]) subdivides into the metencephalon (blue) and myelencephalon (pink). The areas of brain that each of these becomes are listed and color-coded onto a sagittal MRI (right).
Fetal (9–23 Weeks)
By the early fetal phase (Figure 2), limbic structures and their associated tracts are developing rapidly, suggesting early establishment of fronto-limbic connectivity.8 Myelination begins in subcortical regions.12 During this phase, thalamocortical axons arrive in the subplate in sensory and association areas, where they form temporary synapses (Figure 2).7 Interhemispheric (callosal) connections are present below the subplate. Proliferation and migration of neurons to cortex peak.12,13 The mature neocortex is a complex laminar structure that requires the coordinated birth and migration of waves of neuronal precursors. The six layers are laid down in what is often described as an “inside-out” pattern. Neurons destined to become pyramidal cells first migrate to the deepest layers immediately adjacent to the cortical plate. Later-born waves of neurons migrate farther, to the more superficial layers. In contrast, most interneurons originate in the medial and lateral ganglionic eminence and migrate parallel to the cortical surface (tangentially) into cortex and thalamus.12,13 Interneurons and their pyramidal neuron targets must arrive at the same time in the cortex to establish correct connections.14,15 In general, the primary cortices (motor, visual, etc.) develop first, and areas supporting higher cortical function develop later.
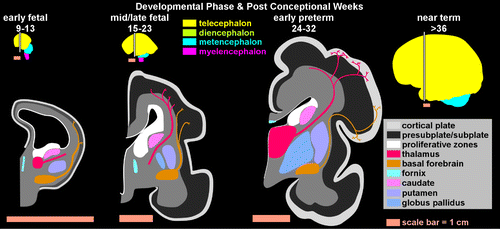
COVER and FIGURE 2. Major phases in prenatal development of the cerebrum are illustrated on schematics of coronal brain sections.2–8 The approximate locations of sections are indicated on drawings of brains color-coded as in Figure 1. Note that the change in shape of the cerebral hemispheres, although often described as rotation, is actually a result of cortical expansion, with little movement of the frontal and temporal poles. In the early fetal phase (left) subcortical nuclei and their associated fiber tracts are already well along in development. Axons from brainstem (not illustrated) and basal forebrain (gold) have made synaptic contact in the regions above the cortical plate (marginal zone, not illustrated) and below the cortical plate (presubplate or subplate, dark gray), whereas most thalamocortical axons (red) have not yet passed through the cortiocostriatal junction. Thalamocortical axons make synaptic contact in the subplate in the midfetal phase (middle). All of these axons wait in the subplate until the early preterm phase (right), when they invade the cortical plate and establish synaptic contacts.
Preterm (24–36 Weeks) and Near-Term (36–41 Weeks)
Axons from subcortical structures (e.g., thalamus, basal forebrain) that have been waiting in the subplate penetrate the cortical plate during the early preterm phase (Figure 2).7 Thalamocortical axons form synapses onto layer IV and III neurons and become sensory driven, providing the basis for sensory-expectant development. The primary sulci develop at this time, and myelination of cortical pathways begins. From this point to term the long intrahemispheric (association) and interhemispheric (callosal) connections are established, gyrification increases, and the secondary sulci develop.
Term and Postnatal
At term and during the early postnatal period, the short cortico-cortical connections are made and the tertiary sulci develop, resulting in the characteristic highly infolded appearance of the cerebral cortex. Myelination peaks during the first postnatal year, but continues for decades. It progresses from inferior to superior, from caudal to rostral, and from central to peripheral. Sensory pathways become myelinated before motor, projection fibers before association fibers, and occipito-parietal regions before temporo-frontal regions.
Pediatric Brain Development and Neuropsychiatric Illness
One of the biggest challenges in studying pediatric neuropsychiatric illness is establishing what is normal as a basis for comparisons. The process of childhood development is both variable and unpredictable. Given the numerous tasks of development (e.g., fine and gross motor coordination, language development, logical thought-processing, emotional stability, impulse-control), it is not surprising that the course of normal development is quite heterogeneous. Moreover, many symptoms that would be considered pathologic in adults may be features of normal development in children. For example, young children (toddlers, preschool age) may exhibit aggressive outbursts because of their limited capacity for impulse-control. Modulating aggression by developing verbal strategies of problem-solving is an important task of normal development. School-age children may exhibit separation anxiety in the absence of psychiatric illness as they struggle with self-confidence and self-efficacy in the face of social and academic demands outside of the umbrella of parental influence and security. Latency-age children (age 7–11 years) may exhibit variations of obsessive-compulsive behavior. As children learn cause-and-effect, order, and rules, they may appear compulsively inflexible and rigid when it comes to tolerating transgressions of others or insisting upon “fairness.” Rapidly-changing moods in adolescents may reflect a normal course of emotional maturation, whereas such mood-swings would be considered pathologic later in life.
Despite the wide range of normal behavior, there are some developmental tasks that all humans must accomplish. Brain development is dependent upon neural activity. Synaptic connections develop if neurons are concurrently active; hence, the adage, “Nerves that fire together, wire together.” The most active neurons or neural networks in the brain become more efficient; the least active neurons are likely to be pruned. Experience expectant development refers to periods during which an experience (or its absence) has a marked impact on the neural organization underlying a particular skill or competence. In other words, the brain expects to receive a certain kind of sensory input (e.g., patterned visual information, language) within a critical time-frame (sensitive period). In the absence of appropriate input, neurons of specialized function may not undergo consolidated development, and that function may be permanently absent or impaired. A well-known example is that kittens deprived of visual input in the first 3 months have permanently impaired vision.16 Finely tuned sound/pitch discrimination appears to be enhanced if children are exposed to a variety of sound pitches before the age of seven.17 Foreign-language learning that begins in early childhood may be better preserved later in life.18 One could well conjecture that sensitive periods of development also apply to more complex human functions, such as impulse-control, sustained attention, mood-regulation, and adaptability.
Younger animals' brains are primed to receive information and respond with brain growth much more readily than in older animals' brains. Neurogenesis is particularly enhanced by enriched environments, new learning, and voluntary exercise. It is inhibited by aging, sleep-deprivation, and stress. A recent study assessing the impact of enhanced environments on preterm infants found that the enhanced-environment group had robust improvements in self-regulation, autonomic stability, and EEG normalization. Also, they had MRI findings of more mature tract development in the internal capsules 9 months later.19 In contrast, the deleterious impact of deprived environments or pathological stress should not be underestimated. Acute stressors trigger physiologic activation (e.g., glucocorticoid release) to ensure the survival of the organism, but to the temporary detriment of systems controlling growth and recuperation. If the brain shifts to a protective mode in order to accomplish self-preservation, then neurogenesis is sacrificed. Frequent activation of the stress response tilts the organism toward consuming resources without sufficient recovery.20 Severe early neglect affects the development of cortico-limbic circuits involved in emotion and stress responses.21 Significant anatomic brain changes can be seen in the context of stress and deprived environments. Post-institutionalized children have larger amygdalae, and size corresponds to the duration of institutional care.22 Furthermore, the neurodevelopmental sequelae of stress may persist over the long term. One study reports that exposure to childhood sexual abuse is associated with a decreased hippocampal volume and a smaller corpus callosum in young adults.23 However, on a positive note, the physiologic stress-response may be reduced by the presence of a secure attachment figure. Toddlers in secure attachment relationships do not show pathologic elevations in cortisol to distress-eliciting events, whereas toddlers in insecure attachment relationships have higher cortisol levels.20
Although specifying the course of normal brain development is a challenge in studying pediatric neuropsychiatric illness, general principles of brain growth as well as new anatomic MRI studies have led to remarkable insights into variable maturation of different brain regions.24 Much of brain development involves cycles of cell growth, synaptogenesis, then pruning and remodeling. Cortical cell numbers seem to plateau soon after birth, but the volume of gray matter may increase until puberty, then decrease.25,26 Formation of connections between neurons and myelination both continue to increase throughout childhood and early adulthood.27 One of the last regions of the brain to become fully myelinated is the dorsolateral prefrontal cortex, an important region of the brain implicated in tasks of ethical decision-making.28
Perhaps the most notable early-onset neuropsychiatric illness in pediatrics is attention-deficit hyperactivity disorder (ADHD). ADHD is a disorder of impaired impulse-control, distractibility, and psychomotor overactivity. There is evidence that baseline caudate volume is smaller in boys with ADHD than in control subjects, and that it does not decrease in size as would be expected, suggesting impairment in the process of pruning and thus becoming more functionally efficient.29 Also, one study has reported reversed caudate asymmetry in boys with ADHD, specifically, a smaller left caudate head.30 The implication is that caudate dysfunction can portend neuropsychiatric illness early in life.
The caudate nucleus also seems to be involved in Tourette's syndrome, another neuropsychiatric illness that usually emerges in school-aged children. Tics are fragments of motor behaviors that escape inhibitory control. Tourette's syndrome may be understood as a model of impaired impulse-control and resultant compensatory response within cortical-striatal-thalamo-cortical circuits. One theory is that the caudate has impaired inhibitory function, and the dorsal prefrontal cortex compensates with increased activity in order to “suppress” tics. If this increased activity leads to a plastic response, the region gets larger. If the plastic response does not occur, it remains small, and more severe tics are observed clinically.31 However, tic severity in childhood does not predict tic severity in adults. Instead, a smaller caudate volume in childhood predicts tic severity in adults, suggesting that structural imaging in adulthood may be measuring compensatory changes.32 Typically developing mesial temporal lobe structures such as the amygdala and the hippocampus increase in size through adolescence, and may not mature fully until adulthood.26,33 In Tourette's syndrome, increases in the dentate and CA3 subfields correlate with symptom improvement. However, increases in the medial body of the hippocampus and posterior surface of the amygdala correlate with more severe symptoms.34 This may be another instance in which structural-imaging studies done in adults identify compensatory changes rather than abnormalities underlying initial symptom development.
Adult Neuropsychiatric Illness
As described previously, neurons must migrate from the subventricular zone to their final locations to build the cerebral cortex. The machinery required for neuron migration includes cytoskeletal components, with the centrosome serving as an anchor for microtubules that project to the leading edge of the migrating neuron. Once the leading edge is attached (either to the pial surface or to a radial glial scaffold), the contraction of microtubules pulls the centrosome and the nucleus toward the leading process. There is an extensive extra- and intracellular signaling network regulating neuron migration (e.g., reelin/Wnt/Dab pathway, which signals through GSK3β, as well as netrins, semaphorins, slit receptors and integrins). There are also protein complexes at the tip of microtubule and the centrosome that control microtubule polymerization and depolymerization (e.g., Lis1, Ndel, and Disc1).15 Mutations in some of these genes produce severe developmental brain defects such as lissencephaly.35
Smaller changes in these centrosomic or microtubule-regulatory proteins may be responsible for more subtle defects in cortical architecture, as are seen in schizophrenia. Abnormalities associated with schizophrenia include misplaced and smaller neurons in both cortex and hippocampus in the absence of evidence for neurodegeneration (e.g., no significant gliosis).36,37 The entorhinal cortex shows similar abnormalities, with decreased cell density and abnormal clustering in layers I/II.38–40 Molecular markers of neurons, synapses and dendrites (e.g., N-acetyl-aspartate,41 SNAP-25,42 complexin II,43 microtubule associated proteins44) are found at lower levels in multiple brain regions in schizophrenia. There are decreased numbers of GABA-ergic interneurons and interneuron markers in the prefrontal cortex, with more pyramidal cells in deeper layers.45 NADP-diaphorase also identifies a shifted neuron laminar distribution, with reduced density in superficial layers and increased density in deeper layers.46,47
Neural net simulations have been used to better understand the possible functional results of alterations in cortical architecture. Simulations utilizing the cortical abnormalities found in schizophrenia show aberrant function that is similar to psychotic symptoms.48 Reduced numbers of parvalbumin-expressing cortical interneurons in schizophrenia diminishes gamma oscillations necessary for proper cortical circuit function.49,50 This is hypothesized to be a core functional module also disrupted by NMDA hypofunction in schizophrenia.51 Similar deficits are seen in animal models for schizophrenia.52 Understanding the mechanisms of abnormal cortical development, and the associated functional impact of the altered cortical histology, is critical to illuminating the pathophysiology of schizophrenia and psychosis, and has the potential to identify novel treatment targets.
Conclusion
The evidence suggests that the human brain has clear developmental trajectories that may have important origins early in life. It may be intuitive to posit that humans, with highly developed association areas in the prefrontal cortex, would have sensitive periods of development early in life that may serve as precursors for cognitive, emotional, and behavioral patterns later in life. Stress and trauma could be key antecedents that “hard-wire” vulnerability for psychiatric illness. As a pediatric perspective is more widely investigated, a better understanding of the natural history of neuropsychiatric illness may emerge. More importantly, it may be possible to develop treatment strategies that serve to change maladaptive developmental patterns and return children, adolescents, and perhaps even adults to a normalized developmental course.
1. : The Human Brain. St. Louis, Mosby, 2002Google Scholar
2. : Correlation between the sequential ingrowth of afferents and transient patterns of cortical lamination in preterm infants. Anat Rec 2002; 267:1–6Crossref, Medline, Google Scholar
3. : White and gray matter development in human fetal, newborn, and pediatric brains. Neuroimage 2006; 33:27–38Crossref, Medline, Google Scholar
4. : The development of cerebral connections during the first 20–45 weeks' gestation. Semin Fetal Neonatal Med 2006; 11:415–422Crossref, Medline, Google Scholar
5. : Anatomical characterization of human fetal brain development with diffusion tensor magnetic resonance imaging. J Neurosci 2009; 29:4263–4273Crossref, Medline, Google Scholar
6. : Insights from in vitro fetal magnetic resonance imaging of cerebral development. Semin Perinatol 2009; 33:220–233Crossref, Medline, Google Scholar
7. : The development of the subplate and thalamocortical connections in the human foetal brain. Acta Paediatr 2010; 99:1119–1127Crossref, Medline, Google Scholar
8. : Development of axonal pathways in the human fetal fronto-limbic brain: histochemical characterization and diffusion tensor imaging. J Anat 2010; 217:400–417Crossref, Medline, Google Scholar
9. : Functional imaging of developmental and adaptive changes in neurocognition. Neuroimage 2006; 30:679–691Crossref, Medline, Google Scholar
10. : Defining clinical phenotypes of juvenile mania. Am J Psychiat 2003; 160:430–437Crossref, Medline, Google Scholar
11. : Neuroimaging studies of normal brain development and their relevance for understanding childhood neuropsychiatric disorders. J Am Acad Child Adolesc Psychiatry 2008; 47:1233–1251Crossref, Medline, Google Scholar
12. : Normal development of brain circuits. Neuropsychopharmacology 2010; 35:147–168Crossref, Medline, Google Scholar
13. : Development of the human cerebral cortex: Boulder Committee revisited. Nat Rev Neurosci 2008; 9:110–122Crossref, Medline, Google Scholar
14. : Life is a journey: a genetic look at neocortical development. Nat Rev Genet 2002; 3:342–355Crossref, Medline, Google Scholar
15. : Trekking across the brain: the journey of neuronal migration. Cell 2007; 128:29–43Crossref, Medline, Google Scholar
16. : The period of susceptibility to the physiological effects of unilateral eye closure in kittens. J Physiol 1970; 206:419–436Crossref, Medline, Google Scholar
17. : Effect of frequency ratio on infants' and adults' discrimination of simultaneous intervals. J Exp Psychol Hum Percept Perform 1997; 23:1427–1438Crossref, Medline, Google Scholar
18. : Preserved implicit knowledge of a forgotten childhood language. Psychol Sci 2009; 20:1064–1069Crossref, Medline, Google Scholar
19. : Early experience alters brain function and structure. Pediatrics 2004; 113:846–857Crossref, Medline, Google Scholar
20. : The neurobiology of stress and development. Annu Rev Psychol 2007; 58:145–173Crossref, Medline, Google Scholar
21. : Bringing basic research on early experience and stress neurobiology to bear on preventive interventions for neglected and maltreated children. Dev Psychopathol 2006; 18:651–677Crossref, Medline, Google Scholar
22. : Prolonged institutional rearing is associated with atypically large amygdala volume and difficulties in emotion regulation. Dev Sci 2010; 13:46–61Crossref, Medline, Google Scholar
23. : Preliminary evidence for sensitive periods in the effect of childhood sexual abuse on regional brain development. J Neuropsychiatry Clin Neurosci 2008; 20:292–301Link, Google Scholar
24. : Structural MRI of pediatric brain development: what have we learned and where are we going? Neuron 2010; 67:728–734Crossref, Medline, Google Scholar
25. : Brain development during childhood and adolescence: a longitudinal MRI study. Nat Neurosci 1999; 2:861–863Crossref, Medline, Google Scholar
26. : Anatomical MRI of the developing human brain: what have we learned? J Am Acad Child Adolesc Psychiatry 2001; 40:1012–1020Crossref, Medline, Google Scholar
27. : Brain development in healthy, hyperactive, and psychotic children. Arch Neurol 2002; 59:1244–1248Crossref, Medline, Google Scholar
28. : The neural processing of moral sensitivity to issues of justice and care. Neuropsychologia 2007; 45:755–766Crossref, Medline, Google Scholar
29. : Toward a pathophysiology of attention-deficit/hyperactivity disorder. Clin Pediatr (Phila) 1997; 36:381–393Crossref, Medline, Google Scholar
30. : Components of attention in children with complex partial seizures with and without ADHD. Epilepsia 1999; 40:211–215Crossref, Medline, Google Scholar
31. : A developmental fMRI study of self-regulatory control in Tourette's syndrome. Am J Psychiatry 2007; 164:955–966Crossref, Medline, Google Scholar
32. : Basal ganglia volumes in patients with Gilles de la Tourette syndrome. Arch Gen Psychiatry 2003; 60:415–424Crossref, Medline, Google Scholar
33. : Developmental trajectories of brain volume abnormalities in children and adolescents with attention-deficit/hyperactivity disorder. JAMA 2002; 288:1740–1748Crossref, Medline, Google Scholar
34. : Morphologic features of the amygdala and hippocampus in children and adults with Tourette syndrome. Arch Gen Psychiatry 2007; 64:1281–1291Crossref, Medline, Google Scholar
35. : The cellular roles of the lissencephaly gene LIS1, and what they tell us about brain development. Genes Dev 2006; 20:1384–1393Crossref, Medline, Google Scholar
36. : Gliosis in schizophrenia. Biol Psychiatry 1988; 24:727–731Crossref, Medline, Google Scholar
37. : The neuropathology of schizophrenia: a critical review of the data and their interpretation. Brain 1999; 122:593–624Crossref, Medline, Google Scholar
38. : Prenatal developmental disturbances in the limbic allocortex in schizophrenics. J Neural Transm 1986; 65:303–326Crossref, Medline, Google Scholar
39. : Some cytoarchitectural abnormalities of the entorhinal cortex in schizophrenia. Arch Gen Psychiatry 1991; 48:625–632Crossref, Medline, Google Scholar
40. : Deficits in small interneurons in prefrontal and cingulate cortices of schizophrenic and schizoaffective patients. Arch Gen Psychiatry 1991; 48:996–1001Crossref, Medline, Google Scholar
41. : Measurement of brain metabolites by 1H magnetic resonance spectroscopy in patients with schizophrenia: a systematic review and meta-analysis. Neuropsychopharmacology 2005; 30:1949–1962Crossref, Medline, Google Scholar
42. : SNAP-25 deficit and hippocampal connectivity in schizophrenia. Cereb Cortex 1998; 8:261–268Crossref, Medline, Google Scholar
43. : Preferential involvement of excitatory neurons in medial temporal lobe in schizophrenia. Lancet 1998; 352:1669–1673Crossref, Medline, Google Scholar
44. : Abnormal expression of two microtubule-associated proteins (MAP2 and MAP5) in specific subfields of the hippocampal formation in schizophrenia. Proc Natl Acad Sci U S A 1991; 88:10850–10854Crossref, Medline, Google Scholar
45. : A cross-study meta-analysis and three-dimensional comparison of cell counting in the anterior cingulate cortex of schizophrenic and bipolar brain. Schizophr Res 2005; 73:79–89Crossref, Medline, Google Scholar
46. : Altered distribution of nicotinamide-adenine dinucleotide phosphate-diaphorase cells in frontal lobe of schizophrenics implies disturbances of cortical development. Arch Gen Psychiatry 1993; 50:169–177Crossref, Medline, Google Scholar
47. : Distorted distribution of nicotinamide-adenine dinucleotide phosphate-diaphorase neurons in temporal lobe of schizophrenics implies anomalous cortical development. Arch Gen Psychiatry 1993; 50:178–187Crossref, Medline, Google Scholar
48. : Neural network models of schizophrenia. Neuroscientist 2001; 7:441–454Crossref, Medline, Google Scholar
49. : A loss of parvalbumin-containing interneurons is associated with diminished oscillatory activity in an animal model of schizophrenia. J Neurosci 2009; 29:2344–2354Crossref, Medline, Google Scholar
50. : Parvalbumin neurons and gamma rhythms enhance cortical circuit performance. Nature 2009; 459:698–702Crossref, Medline, Google Scholar
51. : Circuit-based framework for understanding neurotransmitter and risk gene interactions in schizophrenia. Trends Neurosci 2008; 31:234–242Crossref, Medline, Google Scholar
52. : A neonatal ventral hippocampal lesion causes functional deficits in adult prefrontal cortical interneurons. J Neurosci 2008; 28:12691–12699Crossref, Medline, Google Scholar