Effect of Functional BDNF and COMT Polymorphisms on Symptoms and Regional Brain Volume in Frontotemporal Dementia and Corticobasal Syndrome
Abstract
Objective:
The authors examined the effects of two common functional polymorphisms—brain-derived neurotrophic factor (BDNF) Val66Met and catechol-O-methyltransferase (COMT) Val158Met—on cognitive, neuropsychiatric, and motor symptoms and MRI findings in persons with frontotemporal lobar degeneration (FTLD) syndromes.
Methods:
The BDNF Val66Met and COMT Val158Met polymorphisms were genotyped in 174 participants with FTLD syndromes, including behavioral variant frontotemporal dementia, primary progressive aphasia, and corticobasal syndrome. Gray matter volumes and scores on the Delis-Kaplan Executive Function System, Mattis Dementia Rating Scale, Wechsler Memory Scale, and Neuropsychiatric Inventory were compared between allele groups.
Results:
The BDNF Met allele at position 66 was associated with a decrease in depressive symptoms (F=9.50, df=1, 136, p=0.002). The COMT Val allele at position 158 was associated with impairment of executive function (F=6.14, df=1, 76, p=0.015) and decreased bilateral volume of the head of the caudate in patients with FTLD (uncorrected voxel-level threshold of p<0.001). Neither polymorphism had a significant effect on motor function.
Conclusions:
These findings suggest that common functional polymorphisms likely contribute to the phenotypic variability seen in patients with FTLD syndromes. This is the first study to implicate BDNF polymorphisms in depressive symptoms in FTLD. These results also support an association between COMT polymorphisms and degeneration patterns and cognition in FTLD.
A range of phenotypes, from behavior and language symptoms to motor neuron disease, can be observed in patients with frontotemporal lobar degeneration (FTLD), even across patients with the same pathogenic mutation (1). There is accumulating evidence that functional polymorphisms, including in transmembrane protein 106b (TMEM106B) (2), catechol-O-methyltransferase (COMT) (3), and the dopamine receptor D4 (DRD4) (4), affect phenotype in FTLD-spectrum disorders. In this study, we examined the effects of common functional polymorphisms in two genes—brain-derived neurotrophic factor (BDNF) and COMT—on clinical and MRI findings in patients with frontotemporal dementia (FTD) and corticobasal syndrome.
BDNF is a member of the neurotrophin family of neuron growth factors (5). BDNF contains a functional polymorphism (rs6265) that results in a valine versus methionine substitution at codon 66, termed the Val66Met polymorphism. The Met allele is associated with decreased BDNF activity (6). BDNF is involved in hippocampus-mediated learning and long-term potentiation (7–10). BDNF appears to be involved in the pathogenesis of Huntington’s disease (11), major depressive disorder (12, 13), and the recovery of executive function after brain injury (14). BDNF genotype appears to affect the volume of several brain regions in healthy subjects, including the hippocampus, lateral temporal lobe, prefrontal cortex, and cingulate cortex (15, 16).
COMT regulates dopamine in the prefrontal cortex by converting released dopamine to 3-methoxytyramine (17–19). COMT has a common functional methionine (Met) for valine (Val) substitution at codon 158, referred to as the Val158Met polymorphism (rs4680). The enzyme in individuals with the Met/Met genotype has three to four times lower activity than the enzyme in individuals with the Val/Val genotype (20). Thus, individuals with the Met allele have greater amounts of available dopamine. Individuals with the Met allele demonstrate superior performance on tests of frontal cognitive function in an allele dose-dependent fashion (21–29). The COMT genotype appears to affect the symptom presentation of Alzheimer’s disease (30), schizophrenia (26, 31, 32), attention deficit hyperactivity disorder (33), and brain injury (34). The COMT genotype has also been shown to affect gray matter volume in dopamine-innervated brain regions in patients with dementia, including FTLD-spectrum illnesses (3). Studies have shown that functional polymorphisms in the DRD4 gene that reduce dopamine (DA) tone are associated with greater degeneration of brain regions with high DA receptor density and increased apathy in patients with FTD (4), suggesting that changes in DA may affect both neurodegeneration and symptoms in FTLD syndromes.
In the present study, we assessed the effects of the BDNF Val66Met and COMT Val158Met polymorphisms on cognitive, neuropsychiatric, and motor symptoms and regional brain volumes on MRI in 174 patients with FTLD-spectrum illness. Based on previous findings for healthy control subjects and patients with dementia, we hypothesized that the BDNF Met allele would be associated with memory dysfunction and that the COMT Val allele would be associated with executive and motor dysfunction.
Methods
Participants
A total of 174 patients were genotyped as follows: behavioral variant FTD (bvFTD), N=82; nonfluent variant primary progressive aphasia, N=19; semantic variant primary progressive aphasia (PPA), N=4; FTD-motor neuron disease, N=5; and corticobasal syndrome, N=64. Participants were assessed as part of an ongoing research study on FTLD-spectrum illnesses in the Cognitive Neuroscience Section of the National Institute of Neurological Disorders and Stroke (NINDS) of the National Institutes of Health (NIH). They were either self-referred or referred by outside clinicians. Patients arrived at NIH with a caregiver and were diagnosed by standard clinical criteria in an evaluation by a neurologist (E.M.W.), psychiatrist (E.D.H), and neuropsychologist (J.G.) (35–37). Patients also met updated criteria for probable bvFTD (38). The updated terminology is used in this study. Patients, accompanied by their caregivers, underwent approximately 1 week of extensive neuropsychological and imaging studies. Approximately 6% of this patient cohort had an autosomal dominant mutation known to result in FTD, including mutations in progranulin, microtubule-associated protein tau, and C9orf72 (39). The remainder of patients had sporadic illness. While most of the patients have not yet come to autopsy, those patients with a clinical diagnosis of corticobasal syndrome who later died and underwent autopsy (N=15) demonstrated roughly equal pathological diagnoses for Alzheimer’s disease (N=7) and CBD (N=8).
We required all participants to have an assigned durable power of attorney before enrollment in the study, and the assigned individuals provided written, informed surrogate consent for the patients to enter the study, and all patients provided assent. All aspects of the study and the consent procedure were approved by the NINDS Institutional Review Board. Demographic and clinical characteristics of the study participants are presented in Table 1.
Characteristic | FTD and PPA | CBS | COMT Val+ | COMT Val− | Correlation with COMT Val dose | p | BDNF Met+ | BDNF Met− | Correlation with BDNF Met dose | p |
---|---|---|---|---|---|---|---|---|---|---|
Gender (N) | ||||||||||
Male | 63 | 36 | 96 | 23 | 92 | 4 | ||||
Female | 47 | 28 | 53 | 21 | 70 | 3 | ||||
Age at onset (years; mean) | 55.9 | 59.1 | 57.2 | 57.0 | 0.04 | 60.0 | 62.9 | −0.00 | ||
Age at onset (years; SD) | 8.0 | 7.9 | 8.2 | 8.2 | 7.9 | 4.1 | ||||
Handedness (N) | ||||||||||
Right-handed | 89 | 57 | 106 | 36 | 136 | 5 | ||||
Left-handed | 14 | 4 | 13 | 5 | 16 | 2 | ||||
Ambidextrous | 1 | 1 | 2 | 0 | 2 | 0 | ||||
Education (years; mean) | 15.8 | 15.0 | 15.4 | 15.6 | −0.05 | 15.5 | 14.4 | 0.11 | ||
Education (years; SD) | 2.7 | 2.6 | 2.8 | 2.7 | 2.7 | 3.6 | ||||
Composite scores | ||||||||||
Composite executive measure (mean) | 0.01 | −0.02 | −0.06 | 0.21 | −0.21 | 0.08* | −0.01 | 0.16 | −0.01 | 0.97 |
Composite executive measure (SD) | 0.41 | 0.51 | 0.40 | 0.48 | 0.45 | 0.56 | ||||
Composite memory measure (mean) | 17.15 | 18.60 | 17.49 | 18.44 | −0.05 | 0.58 | 17.49 | 21.67 | −0.04 | 0.64 |
Composite memory measure (SD) | 7.19 | 6.79 | 6.89 | 7.16 | 7.05 | 7.61 | ||||
Composite motor measure (mean) | 0.15 | −0.24 | −0.19 | 0.05 | −0.07 | 0.53 | −0.13 | −0.01 | −0.08 | 0.51 |
Composite motor measure (SD) | 0.92 | 0.78 | 0.87 | 0.79 | 0.84 | 1.05 | ||||
Neuropsychiatric Inventory score | ||||||||||
Total mean | 26.91 | 10.53 | 20.55 | 22.14 | −0.03 | 0.76 | 20.47 | 23.33 | −0.04 | 0.67 |
Total SD | 16.75 | 10.48 | 16.85 | 16.90 | 16.99 | 15.02 | ||||
Delusions mean | 0.33 | 0.08 | 0.21 | 0.34 | −0.08 | 0.29 | 0.23 | 0.00 | 0.01 | 0.25 |
Delusions SD | 1.11 | 0.44 | 1.00 | 0.80 | 0.92 | 0.00 | ||||
Hallucinations mean | 0.32 | 0.00 | 0.27 | 0.00 | 0.11 | 0.20 | 0.20 | 0.00 | 0.13 | 0.12 |
Hallucinations SD | 1.15 | 0.00 | 0.27 | 0.00 | 0.96 | 0.00 | ||||
Agitation mean | 2.68 | 1.22 | 2.05 | 2.63 | −0.11 | 0.21 | 2.12 | 2.50 | −0.07 | 0.42 |
Agitation SD | 3.07 | 1.92 | 2.73 | 3.12 | 2.88 | 2.35 | ||||
Depression mean | 1.18 | 1.78 | 1.48 | 1.29 | −0.04 | 0.62 | 1.28 | 4.50 | −0.22 | 0.01** |
Depression SD | 2.37 | 2.79 | 2.77 | 1.95 | 2.27 | 5.93 | ||||
Anxiety mean | 2.99 | 1.51 | 2.44 | 2.77 | −0.07 | 0.39 | 2.48 | 1.67 | 0.05 | 0.56 |
Anxiety SD | 3.37 | 2.32 | 3.12 | 3.30 | 3.17 | 2.42 | ||||
Euphoria mean | 1.71 | 0.31 | 1.10 | 1.57 | −0.04 | 0.65 | 1.25 | 1.33 | 0.05 | 0.57 |
Euphoria SD | 2.58 | 1.24 | 2.10 | 2.89 | 2.30 | 3.27 | ||||
Apathy mean | 6.59 | 2.47 | 5.17 | 5.20 | 0.01 | 0.95 | 4.98 | 7.17 | −0.03 | 0.70 |
Apathy SD | 3.90 | 3.14 | 4.22 | 4.06 | 4.20 | 3.25 | ||||
Disinhibition mean | 3.97 | 1.04 | 2.93 | 3.06 | 0.03 | 0.73 | 3.03 | 1.67 | 0.001 | 0.98 |
Disinhibition SD | 4.11 | 2.15 | 3.89 | 3.75 | 3.90 | 3.20 | ||||
Irritability mean | 3.04 | 1.02 | 2.30 | 2.60 | −0.05 | 0.55 | 2.25 | 2.33 | −0.10 | 0.27 |
Irritability SD | 3.60 | 2.24 | 3.42 | 3.26 | 3.34 | 3.67 | ||||
Motor behavior mean | 4.36 | 0.92 | 3.19 | 3.20 | 0.06 | 0.47 | 3.23 | 2.17 | 0.07 | 0.40 |
Motor behavior SD | 4.22 | 1.96 | 3.89 | 4.32 | 4.00 | 3.25 |
TABLE 1. Demographic characteristics of the study participants and values for cognitive and behavioral measures by genotypea
Genotyping
Peripheral blood samples were collected from patients in 5-ml EDTA tubes. Genomic DNA was extracted from peripheral blood as recommended by the manufacturer (Wizard Genomic DNA Purification Kit, Promega, Madison, Wis.). After quality assessment and quantification using NanoDrop ND-1000 (Thermo Scientific, Waltham, Mass.), 10 ng of DNA samples were genotyped in a 5-µl TaqMan genotyping assay as recommended by the manufacturer (Applied Biosystems, Waltham, Mass.). Primers and probes for the single-nucleotide polymorphism (SNP) rs4680 in the COMT gene and the SNP rs6265 in the BDNF gene were obtained from Applied Biosystems. The assays were run on an ABI PRISM 7900 Sequence Detection System instrument (Applied Biosystems). The genotyping results were analyzed using SDS software version 2.3 (Applied Biosystems). For quality control, 10% of assays were repeated for the genotyping assay, and the results were 100% concordant.
Cognitive, Behavioral, and Motor Analyses
We assessed a broad range of cognition, including all of the neuropsychological tests previously examined by Huey et al. (40): the Delis-Kaplan Executive Function System (D-KEFS) (41), the Mattis Dementia Rating Scale (MDRS-2) (42), and the Wechsler Memory Scale (WMS-III) (43). We assessed neuropsychiatric symptoms with the Neuropsychiatric Inventory (NPI) (44). The same subtests were analyzed in the present study as in Huey et al. (40). In pilot testing, many patients experienced difficulty understanding some of the more challenging D-KEFS tests. Therefore, of the nine D-KEFS tests, only five were administered and analyzed: Trail-Making Test (including Parts A and B), Verbal Fluency, Sorting, Twenty Questions, and the Tower test. All primary scaled measures of the D-KEFS tests (excluding measures that are derived from primary measures) were used in the factor analysis. The sample size and ratio of participants to variables satisfies guidelines for principle components analysis (45). To assess motor function, we analyzed the mean number of taps on the Finger Tapping Test (46) and number of pegs placed on the Grooved Pegboard Test for dominant and nondominant hands and the Composite Standard Score on the Test of Oral and Limb Apraxia (TOLA) (47).
Statistical Analyses
To explore the relationship between allele dosage and symptoms, we used an analysis of variance (ANOVA) to compare the scores on our cognitive and behavioral symptoms between patients with and without a BDNF Met or COMT Val allele dosage. For primary analyses to test our hypotheses that COMT Val alleles would be associated with worsened executive and motor function and BDNF Met alleles would be associated with worsened memory, composite scores for memory, executive and motor function were compared, followed by subsequent test of the subtests that comprised the composite score. If the ANOVA for the composite measure was not significant when corrected for multiple comparisons of the composite measures, we did not continue to subtest analyses. The composite scores were calculated as follows: the mean of the D-KEFS factor scores (executive), the mean of the WMS-III standardized scores (memory), and the mean of z-scores of the Finger Tapping, Grooved Pegboard, and TOLA scores (Table 1). To obtain summary scores for the D-KEFS, we performed the same factor analysis (a principal components analysis using Varimax with Kaiser normalization) on the D-KEFS subscores, as specified in Gorno-Tempini et al. (37). To explore components of the composite scores, we performed subsequent correlations between the number of COMT Val and BDNF Met alleles (termed the allele dosage: 0, 1, or 2 per participant) and measures of cognitive, behavioral, and motor symptoms.
Imaging
A 1.5-T GE MRI scanner (GE Medical Systems, Milwaukee, Wis.) and standard quadrature head coil were used to obtain all images. A T1-weighted spoiled gradient echo sequence was used to generate 124 contiguous 1.5-mm-thick axial slices (repetition time=6.1 msec; echo time=min full; flip angle=20°; field of view=240 mm; 124 slices, slice thickness=1.5 mm; matrix size=256×256×124). Voxel-based morphometry analysis of the data was performed with SPM8 and followed the principles outlined by Ridgway et al. (48). Except as noted below, all default SPM options were used. Images were segmented into gray matter, white matter, and CSF. Spatial normalization, segmentation, and modulation were processed using a unified segmentation algorithm (49). This algorithm simultaneously calculated image registration, tissue classification, and bias correction using our participants’ structural MR images combined with the tissue probability maps provided in SPM. The segmented and modulated normalized gray matter images were smoothed with an 8-mm full width at half-maximum Gaussian kernel. An explicit mask encompassing the entire brain was used in the analyses to control for background signal outside the brain. This mask was downloaded from the SPM Anatomical Automatic Labeling toolbox. An explicit absolute threshold of p<0.05 for masking was used in the SPM second-level model interface (50). Total intracranial volume was calculated in SPM8 from the unsmoothed, modulated gray matter, white matter, and CSF images from each patient and used as a nuisance variable to account for the possible effect of varying brain volumes.
Two sets of imaging analyses were performed. First, two whole-brain ANOVAs were performed in SPM comparing two to zero copies of the COMT Val and BDNF Met alleles on gray matter volumes. Clusters surviving an uncorrected p-value threshold <0.001 and a cluster size of 30 voxels were considered significant (3). Second, the MarsBar toolbox was used to create regions of interest from clusters found to be significant on the whole brain analysis, as in Gennatas et al. (3). Mean gray matter intensity values were extracted from these regions of interest and used for correlations with our cognitive and behavioral measures.
Results
Demographic Characteristics
We examined a total of 174 participants with FTLD-spectrum illnesses. Table 1 summarizes the results of our analysis of the relationship between COMT Val and BDNF Met allele dosage and composite cognitive, behavioral, and motor measures in patients with FTLD as well as demographic data for the cohort. There were no statistically significant differences in the demographic characteristics of the FTD and PPA versus corticobasal syndrome groups. Therefore, in analyzing the effect of BDNF and COMT polymorphisms, these groups were combined. There was no effect of BDNF or COMT polymorphism on age at onset or other demographic variables (Table 1).
Cognitive, Behavioral, and Motor Analyses
In the principal components analysis, the measures of the correlation between the variables were good, indicating that a principle components analysis was appropriate (the Kaiser-Meyer-Olkin measure was 0.83; Barlett’s Test of Sphericity: χ2=1337.71, df=190, p<0.001). Five components had an eigenvalue >1.0, accounting for 75.5% of the total value. Each of the five components corresponded to a single D-KEFS subtest (i.e., each of the subtests was significantly loaded; absolute value >0.70 on the rotated component matrix). There was a significant effect of the COMT Val allele on our composite executive function measure (F=6.14, df=1, 76, p=0.015) but no significant effect of the COMT Val allele on the memory or motor composite measures. Correlations between the D-KEFS subtests and allele dosage revealed that the significant effect of COMT on executive function was due to its effect on the Sorting test (r=0.22, two-tailed p=0.049, corresponding to a Cohen’s d of 0.45 and r2 of 0.05). None of the other correlations between the D-KEFS subtest factor scores and COMT or BDNF allele dosage were significant. There was a significant effect, surviving correction for multiple comparisons, of the BDNF Met allele only on depressive symptoms as measured by the NPI (F=9.50, df=1, 136, p=0.002) (Table 1). The BDNF Met allele did not have a significant effect on the composite motor measure.
Imaging
Two versus zero BDNF alleles did not have a significant effect on gray matter volume in the whole brain analysis. A COMT Val dosage of 2 was significantly associated with reduced gray matter volume in the bilateral caudate nuclei compared with a dose of 0 (Figure 1). Within the areas found to have significantly reduced gray matter volume, gray matter density was negatively correlated with verbal fluency and sorting subtests of the D-KEFS, with the conceptualization subscale of the MDRS-2, and visual memory on the WMS-III. Gray matter density in the right and left caudate was also negatively correlated with the total NPI and several of the NPI subscales, including agitation, euphoria, apathy, disinhibition, and motor behavior (Table 2).
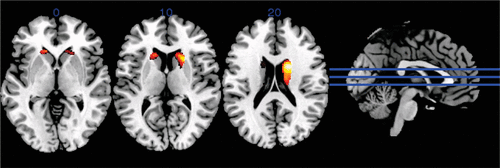
FIGURE 1. Difference between gray matter volume in participants with two compared with zero Val alleles at the catechol-O-methyltransferase Val158Met polymorphisma
a Dark areas show regions of decreased gray matter volume, significant at an uncorrected voxel-level threshold of p<0.001.
Left correlation | Right correlation | |||
---|---|---|---|---|
NPI subscale | r | p | r | p |
Total | −0.322 | <0.000** | −0.146 | 0.050* |
Delusions | 0.041 | 0.329 | 0.054 | 0.280 |
Hallucinations | −0.051 | 0.288 | −0.052 | 0.284 |
Agitation | −0.178 | 0.025* | −0.136 | 0.069 |
Depression | 0.146 | 0.054 | 0.019 | 0.417 |
Anxiety | −0.121 | 0.094 | −0.041 | 0.328 |
Euphoria | −0.358 | <0.000** | −0.300 | <0.000** |
Apathy | −0.318 | <0.000** | −0.116 | 0.102 |
Disinhibition | −0.338 | <0.000** | −0.154 | 0.046* |
Irritability | −0.147 | 0.054 | −0.083 | 0.183 |
Motor behavior | −0.326 | <0.000** | −0.063 | 0.246 |
TABLE 2. Correlations between gray matter density and the Neuropsychiatric Inventory (NPI) in the right and left areas of the caudate nucleusa
Discussion
In patients with FTD and corticobasal syndrome, a greater number of Met alleles at position 66 of BDNF was associated with more depressive symptoms, and more Val alleles at position 158 of COMT was associated with poorer performance on the D-KEFS Sorting task, a test of executive function. We did not find an effect of either polymorphism on motor function. Our findings are consistent with previous studies that have shown associations between the COMT Val158Met and DRD4 polymorphisms and executive function (15, 29) and the BDNF Val66Met polymorphism and depression (12, 13). However, this is the first study, to our knowledge, to implicate BDNF polymorphisms in the clinical presentation of FTLD illnesses. Unexpectedly, the effect is that a greater number of BDNF Met alleles are associated with decreased depression. This is the opposite direction from what has been observed in most previous studies in neurologically intact participants (51, 52), although it is the same direction we observed in our previous study on recovery of executive function after TBI (14). As can be seen in Table 2, the anatomic association between depression and caudate gray matter density is also in the opposite direction of the associations with most of the other neuropsychiatric symptoms (i.e., greater gray matter density is associated with greater depression). However, these results agree with previous findings that suggest that damage to certain brain structures and regions can be protective against internalizing psychiatric symptoms, including depression and anxiety (53–56). Our subsequent analyses showing an association between our cognitive and behavioral measures and the gray matter density in the caudate nuclei further support the relationship between COMT genotype and phenotypic presentation in FTLD (Table 2).
The effect size of BDNF allele dosage on depression, as well as the COMT allele dosage on the Sorting test in patients with FTLD, is medium (Cohen’s d=0.45), accounting for approximately 5% of the variance. This is similar to the amount of variance accounted for by the BDNF Val66Met polymorphism on executive function in the recovery from acute brain injury (6%) (14). However, it is considerably larger than the effects of both of these polymorphisms in healthy subjects (3, 12, 57). This may suggest that these polymorphisms play a larger role in the setting of brain dysfunction than in cognition in healthy subjects.
In our MRI analysis, we found that having two Val alleles at codon 158 of COMT was associated with decreased gray matter volumes only in the bilateral caudate nucleus (Figure 1) compared with zero Val alleles. Gennatas et al. (2012) (3) also found gray matter volume changes associated with increasing COMT Val dose in DA-innervated brain regions including the striatum in patients with dementia, including FTLD. In both studies, associations were observed between cognitive measures, behavioral measures, and gray matter density within DA-innervated brain regions (Table 2). Thus, we can assert with greater confidence the conclusions that were reached by Gennatas et al. (3): increased synaptic DA catabolism associated with the COMT Val allele appears to promote neurodegeneration within DA-innervated brain areas. However, Gennatas et al. (3) found this in several brain structures, whereas we found a significant association only in the bilateral caudate. This difference could be due in part to differences in our patient groups. Gennatas et al. (3) included patients with Alzheimer’s disease, bvFTD, and semantic dementia, whereas our study was limited to patients with FTLD-spectrum illnesses. In addition, our patient group included individuals with corticobasal syndrome, who have more disease involvement of the basal ganglia than other patients with FTLD (58).
The specificity we found of the volume loss related to COMT genotype for the caudate is striking (Figure 1). The caudate is heavily DA-innervated (59), and the caudate and putamen receive extensive afferent projections from the cerebral cortex (60). In corticobasal syndrome, the caudate and putamen show significant tau deposition, but so do several other structures, including the primary motor cortex and the subthalamic nucleus (61). Because all FTLD disorders involve significant cortical degeneration of areas that project to the basal ganglia, one might expect that the caudate, as a major target of those projections, could be particularly affected. Indeed, there is animal evidence that the caudate is more vulnerable to DA depletion than other structures in the basal ganglia (59).
Alzheimer’s disease appears to selectively target the cholinergic neurotransmitter system, and most available treatments for this illness increase available acetylcholine. The brain areas affected in FTD receive extensive dopaminergic and serotonergic projections (62). However, it is not known whether this is an anatomical coincidence (i.e., the disease process targets the frontal and anterior temporal lobes, and these just happen to receive dopaminergic and serotonergic projections) or whether these neurotransmitter systems influence the tropism and progression of the illness. The present study and related studies (3, 4) suggest that the DA system can affect the presentation of the illness and that DA-innervated areas may be particularly vulnerable.
Together, the findings of this study suggest that COMT and BDNF polymorphisms may explain, in part, the phenotypic variability of FTD and corticobasal syndrome. In addition, our results, along with those of other studies, highlight the importance of DA balance in FTLD and support the trial of medications augmenting dopamine in patients with FTD. Indeed, clinical trials of COMT inhibition in patients with FTLD are under way.
1 : Phenotypic heterogeneity of monogenic frontotemporal dementia. Front Aging Neurosci 2015; 7:171Crossref, Medline, Google Scholar
2 : What we know about TMEM106B in neurodegeneration. Acta Neuropathol 2016; 132:639–651Crossref, Medline, Google Scholar
3 : COMT Val158Met genotype influences neurodegeneration within dopamine-innervated brain structures. Neurology 2012; 78:1663–1669Crossref, Medline, Google Scholar
4 : Dopamine receptor D4 (DRD4) polymorphisms with reduced functional potency intensify atrophy in syndrome-specific sites of frontotemporal dementia. Neuroimage Clin 2019; 23:101822Crossref, Medline, Google Scholar
5 : Physiology of the neurotrophins. Annu Rev Neurosci 1996; 19:289–317Crossref, Medline, Google Scholar
6 : The BDNF val66met polymorphism affects activity-dependent secretion of BDNF and human memory and hippocampal function. Cell 2003; 112:257–269Crossref, Medline, Google Scholar
7 : Protein synthesis and neurotrophin-dependent structural plasticity of single dendritic spines. Science 2008; 319:1683–1687Crossref, Medline, Google Scholar
8 : Essential role of BDNF in the mesolimbic dopamine pathway in social defeat stress. Science 2006; 311:864–868Crossref, Medline, Google Scholar
9 : Brain-derived neurotrophic factor val66met polymorphism affects human memory-related hippocampal activity and predicts memory performance. J Neurosci 2003; 23:6690–6694Crossref, Medline, Google Scholar
10 : Brain-derived neurotrophic factor polymorphism Val66Met influences cognitive abilities in the elderly. Genes Brain Behav 2008; 7:411–417Crossref, Medline, Google Scholar
11 : Role of brain-derived neurotrophic factor in Huntington’s disease. Prog Neurobiol 2007; 81:294–330Crossref, Medline, Google Scholar
12 : Impact of the BDNF Val66Met polymorphism on cognition: implications for behavioral genetics. Neuroscientist 2012; 18:439–451Crossref, Medline, Google Scholar
13 : Association of the brain-derived neurotrophic factor Val66Met polymorphism with reduced hippocampal volumes in major depression. Arch Gen Psychiatry 2007; 64:410–416Crossref, Medline, Google Scholar
14 : The role of the Met66 brain-derived neurotrophic factor allele in the recovery of executive functioning after combat-related traumatic brain injury. J Neurosci 2011; 31:598–606Crossref, Medline, Google Scholar
15 : The brain-derived neurotrophic factor val66met polymorphism and variation in human cortical morphology. J Neurosci 2004; 24:10099–10102Crossref, Medline, Google Scholar
16 : Cognitive and magnetic resonance imaging brain morphometric correlates of brain-derived neurotrophic factor Val66Met gene polymorphism in patients with schizophrenia and healthy volunteers. Arch Gen Psychiatry 2006; 63:731–740Crossref, Medline, Google Scholar
17 : Dopamine transporter immunoreactivity in monkey cerebral cortex: regional, laminar, and ultrastructural localization. J Comp Neurol 2001; 432:119–136Crossref, Medline, Google Scholar
18 : Effects of catecholamine uptake blockers in the caudate-putamen and subregions of the medial prefrontal cortex of the rat. Brain Res 2002; 936:58–67Crossref, Medline, Google Scholar
19 : Dopamine uptake through the norepinephrine transporter in brain regions with low levels of the dopamine transporter: evidence from knock-out mouse lines. J Neurosci 2002; 22:389–395Crossref, Medline, Google Scholar
20 : Kinetics of human soluble and membrane-bound catechol O-methyltransferase: a revised mechanism and description of the thermolabile variant of the enzyme. Biochemistry 1995; 34:4202–4210Crossref, Medline, Google Scholar
21 : Effect of COMT Val108/158 Met genotype on frontal lobe function and risk for schizophrenia. Proc Natl Acad Sci USA 2001; 98:6917–6922Crossref, Medline, Google Scholar
22 : Executive subprocesses in working memory: relationship to catechol-O-methyltransferase Val158Met genotype and schizophrenia. Arch Gen Psychiatry 2003; 60:889–896Crossref, Medline, Google Scholar
23 : Catechol O-methyltransferase val158-met genotype and individual variation in the brain response to amphetamine. Proc Natl Acad Sci USA 2003; 100:6186–6191Crossref, Medline, Google Scholar
24 : Genetic and neurochemical modulation of prefrontal cognitive functions in children. Am J Psychiatry 2004; 161:125–132Crossref, Medline, Google Scholar
25 : New evidence of association between COMT gene and prefrontal neurocognitive function in healthy individuals from sibling pairs discordant for psychosis. Am J Psychiatry 2004; 161:1110–1112Crossref, Medline, Google Scholar
26 : Catechol O-methyltransferase Val158Met polymorphism in schizophrenia: differential effects of Val and Met alleles on cognitive stability and flexibility. Am J Psychiatry 2004; 161:359–361Crossref, Medline, Google Scholar
27 : Effect of catechol-O-methyltransferase Val158Met genotype on attentional control. J Neurosci 2005; 25:5038–5045Crossref, Medline, Google Scholar
28 : Catechol O-methyltransferase Val158Met polymorphism is associated with cognitive performance in nondemented adults. J Cogn Neurosci 2005; 17:1018–1025Crossref, Medline, Google Scholar
29 : Tolcapone improves cognition and cortical information processing in normal human subjects. Neuropsychopharmacology 2007; 32:1011–1020 Crossref, Medline, Google Scholar
30 : Catechol-O-methyltransferase gene polymorphism is associated with risk of psychosis in Alzheimer Disease. Neurosci Lett 2004; 370:127–129Crossref, Medline, Google Scholar
31 : Catechol-O-methyltransferase val108/158met genotype predicts working memory response to antipsychotic medications. Biol Psychiatry 2004; 56:677–682Crossref, Medline, Google Scholar
32 : Interaction of COMT (Val(108/158)Met) genotype and olanzapine treatment on prefrontal cortical function in patients with schizophrenia. Am J Psychiatry 2004; 161:1798–1805Crossref, Medline, Google Scholar
33 : Haplotype relative risk study of catechol-O-methyltransferase (COMT) and attention deficit hyperactivity disorder (ADHD): association of the high-enzyme activity Val allele with ADHD impulsive-hyperactive phenotype. Am J Med Genet 1999; 88:497–502Crossref, Medline, Google Scholar
34 : Association of COMT Val158Met genotype with executive functioning following traumatic brain injury. J Neuropsychiatry Clin Neurosci 2005; 17:465–471Link, Google Scholar
35 : Corticobasal degeneration: The syndrome and the disease, in Atypical Parkinsonian Disorders: Clinical and Research Aspects. Edited by Litvan I. Totowa, NJ, Humana Press, 2005, pp 309–334Crossref, Google Scholar
36 : Frontotemporal lobar degeneration: a consensus on clinical diagnostic criteria. Neurology 1998; 51:1546–1554Crossref, Medline, Google Scholar
37 : Classification of primary progressive aphasia and its variants. Neurology 2011; 76:1006–1014Crossref, Medline, Google Scholar
38 : Sensitivity of revised diagnostic criteria for the behavioural variant of frontotemporal dementia. Brain 2011; 134:2456–2477Crossref, Medline, Google Scholar
39 : Characteristics of frontotemporal dementia patients with a Progranulin mutation. Ann Neurol 2006; 60:374–380Crossref, Medline, Google Scholar
40 : Executive dysfunction in frontotemporal dementia and corticobasal syndrome. Neurology 2009; 72:453–459Crossref, Medline, Google Scholar
41 : The Delis-Kaplan Executive Function System: Examiner’s Manual. San Antonio, TX, Psychological Corporation, 2001Crossref, Google Scholar
42 : Mental Status examination for organic mental syndrome in the elderly patient; in Geriatric Psychiatry. Edited by Bellack L, Karusu TB. New York, Grune & Stratton, 1976, pp 77–121Google Scholar
43 : Wechsler Memory Scale Revised. New York, Harcourt Brace Jovanovich, 1987Google Scholar
44 : The Neuropsychiatric Inventory: comprehensive assessment of psychopathology in dementia. Neurology 1994; 44:2308–2314Crossref, Medline, Google Scholar
45 : An Easy Guide to Factor Analysis. London, Routledge, 1994Google Scholar
46 : The Halstead-Reitan Neuropsychological Test Battery: Theory and Interpretation. Tucson, AZ, Neuropsychology Press, 1985Google Scholar
47 : Test of Oral and Limb Apraxia Normed Edition. Chicago, Riverside, 1992Google Scholar
48 : Ten simple rules for reporting voxel-based morphometry studies. Neuroimage 2008; 40:1429–1435Crossref, Medline, Google Scholar
49 : Unified segmentation. Neuroimage 2005; 26:839–851Crossref, Medline, Google Scholar
50 : Why voxel-based morphometry should be used. Neuroimage 2001; 14:1238–1243Crossref, Medline, Google Scholar
51 : Meta-analysis of the BDNF Val66Met polymorphism in major depressive disorder: effects of gender and ethnicity. Mol Psychiatry 2010; 15:260–271Crossref, Medline, Google Scholar
52 : The Val66Met polymorphism of the brain-derived neurotrophic-factor gene is associated with geriatric depression. Neurobiol Aging 2006; 27:1834–1837Crossref, Medline, Google Scholar
53 : Psychiatric symptoms in preclinical behavioural-variant frontotemporal dementia in MAPT mutation carriers. J Neurol Neurosurg Psychiatry 2018; 89:449–455Crossref, Medline, Google Scholar
54 : Distinct regions of prefrontal cortex mediate resistance and vulnerability to depression. J Neurosci 2008; 28:12341–12348Crossref, Medline, Google Scholar
55 : Focal brain damage protects against post-traumatic stress disorder in combat veterans. Nat Neurosci 2008; 11:232–237Crossref, Medline, Google Scholar
56 : Brain regions associated with internalizing and externalizing psychiatric symptoms in patients with penetrating traumatic brain injury. J Neuropsychiatry Clin Neurosci 2016; 28:104–111Link, Google Scholar
57 : Meta-analysis of the cognitive effects of the catechol-O-methyltransferase gene Val158/108Met polymorphism. Biol Psychiatry 2008; 64:137–144Crossref, Medline, Google Scholar
58 : Corticobasal degeneration: a pathologically distinct 4R tauopathy. Nat Rev Neurol 2011; 7:263–272Crossref, Medline, Google Scholar
59 : The metabolic rate and vulnerability of dopaminergic neurons, and adenosine dynamics in the cerebral cortex, nucleus accumbens, caudate nucleus, and putamen of the common marmoset. J Neurol 2000; 247(Suppl 5):V16–V22Crossref, Medline, Google Scholar
60 : Functional architecture of basal ganglia circuits: neural substrates of parallel processing. Trends Neurosci 1990; 13:266–271Crossref, Medline, Google Scholar
61 : Neuropathological features of corticobasal degeneration presenting as corticobasal syndrome or Richardson syndrome. Brain 2011; 134:3264–3275Crossref, Medline, Google Scholar
62 : A systematic review of neurotransmitter deficits and treatments in frontotemporal dementia. Neurology 2006; 66:17–22Crossref, Medline, Google Scholar