Astroglia: Not Just Glue
Astrocytes have been shown to be involved in the regulation of the brain microenvironment, in particular as regards neurotransmitter and ionic homeostasis, metabolic support of neurons, regulation of energy metabolism, synaptic transmission and neuronal excitability, synaptic generation, detoxification, free-radical scavenging, metal sequestration, development and maintenance of the blood-brain barrier, guidance of neuronal migration and immune function. 6
Unlike neurons, astrocytes are not excited electrically, and so were long thought to have no role in communication. 11 One of the most remarkable changes has been the gradual realization that astrocytes modulate synaptic activity both locally and regionally and may well be essential for integration and synchronization of neuronal activity.
Basic Biology of Astroglia
There appears to be an evolutionary pressure increasing the ratio of astroglia to neurons in cortex, perhaps as a function of brain complexity ( Figure 1 ). 12 , 13 Astrocytes within human cortical gray matter also have conformations that differ from lower animals. The classic star-shaped (stellate) astrocytes in humans are both much larger and more complex and diverse in shape ( Figure 1 ). 1 , 13 In addition, there are two forms that are seen only in humans and some lower primates. 1 , 14 – 16 One is the intralaminar astrocyte that resides in cortical layer I and extends long processes (up to 1 mm) down into layers III–IV. The other is the polarized astrocyte that resides in layers V–VI and extends long processes (up to 1 mm) up through the cortical layers. Although the functional significance of these primate-specific astrocytes has not yet been determined, their vertical orientation has lead to the hypothesis that they participate in the modular organization of the cerebral cortex by enhancing the segregation of cortical columns. 14 , 15
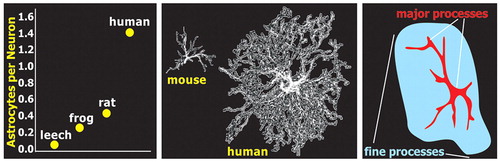
The classic star-shaped (stellate) appearance of astrocytes, as seen with stains for the cytoskeleton such as glial fibrillary acidic protein, results from strong staining only of the thicker processes ( Figure 1 ). In reality, astrocytes have many fine highly branching processes. 1 , 11 , 12 , 17 , 18 These processes make contact with multiple neurons and blood vessels. Studies in both the cortex and hippocampus indicate that each astrocyte forms a local domain, with little or no overlap of processes between adjacent territories ( Figure 2 ). Thus, astrocyte domains provide a highly organized parcellation of gray matter into multiple small compartments, each modulated by a single astrocyte. 12 The dendritic tree of each neuron contacts multiple astrocytes ( Figure 2 ). 18 In mouse cortex, each astrocyte surrounds and envelops an average of four neuronal cell bodies and 300–600 dendrites ( Figure 2 ). While numbers undoubtedly vary, it is estimated that a cortical astrocyte domain in a rat covers ∼90,000 synapses. 1 In contrast, the domains of the much larger human cortical astrocytes are estimated to include ∼2 million synapses. 1

Capillaries commonly do not penetrate an astrocyte’s domain, but rather are positioned at the borders between adjacent domains. 12 Local cerebral blood flow increases in response to increased local neuronal activity (functional hyperemia), assuring an ample supply of the substrates required to support the increased energy demand. The major functional neuroimaging methods all detect some aspect of this process (e.g., blood oxygenation, cerebral blood flow, cerebral glucose uptake). This response is complex in that both local arterioles and their upstream arteries dilate in response to local synaptic activity. 19 In some manner, synaptic activation generates signals that control functional hyperemia. Astrocytes that envelop neurons also make close contact with the capillary network via end-feet and thus are well positioned to participate in both the blood-brain barrier and local regulation of cerebral blood flow. The mechanisms by which this may occur are a subject of active investigation and debate. 19 – 21
The astrocytic processes envelop synapses, forming a physical barrier that limits diffusion of the neurotransmitter away from the synapse. These three elements (presynaptic, postsynaptic, astrocyte) form what is now called the tripartite synapse ( Figure 3 ). 8 , 22 Some models add the extracellular space as a fourth element. 23 Astrocytes maintain the peri-synaptic extracellular environment. They are critical for the rapid removal of the K + that accumulates as a result of neuronal activity. They take up some neurotransmitters, particularly glutamate and γ-aminobutyric acid (GABA), thus assuring prompt termination of action and preventing receptor desensitization. It is estimated that ∼80% of the glutamate released during neurotransmission is taken up by astrocytes. 24 Astrocytes also convert the glutamate to glutamine and release it for uptake into presynaptic terminals where it is converted back to glutamate and released (glutamate-glutamine shuttle). Disruption of these supporting functions may play a role in many brain disorders. Reduced clearance of glutamate, for example, can lead to excitotoxic neuronal injury. 13
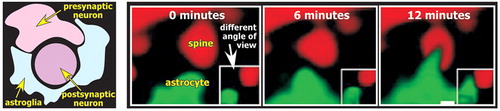
The close contact between astrocytes and neurons provides avenues for communication in both directions. As a result, local neuronal activity modulates astrocytic activity, which in turn modulates local neuronal activity. Gap junctions interconnect astrocytes, thus forming a network (syncytium) that provides an avenue for modulation and synchronization of activity on a much larger scale. The purposes served by gap junctions formed between processes of a single astrocyte (autocellular junctions) are not yet known but may include physical support, enhanced intracellular movement of energy substrates, and coordination of activity within the domain. 1 , 12
Astroglia are responsive to and have specific receptors for many neurotransmitters. 22 A review of in vitro studies utilizing a variety of astrocyte preparations noted that receptors have been identified for norepinephrine, acetylcholine, histamine, serotonin, dopamine, glutamate, aspartate, and GABA. 25 Studies performed in situ and in vivo indicate that expression of receptor types (and subtypes) is quite varied within and across regions as well as during development. 26 Neuronal release of glutamate, for example, activates both ionotropic and metabotropic receptors on astrocytes in addition to glutamate transporters. 24 Stimulation of these glutamate receptors evokes transient calcium increases within the astrocyte (calcium oscillations). In some cases the calcium increase propagates to surrounding astrocytes (calcium wave), although it is not yet certain whether this is a normal part of signaling or a pathophysiological event. 12 , 20 , 22 Interestingly, calcium waves can both increase and decrease neuronal activity.
Astrocytes release many agents including gliotransmitters, neuropeptides, and growth factors. 6 , 8 , 12 The most studied of the gliotransmitters are glutamate, ATP, and D-serine. 6 Even at this early stage of research, the functions that have been identified clearly indicate an active role for astrocytes in modulation of neuronal activity. Astrocytic release of glutamate leads to generation of a slow inward current in surrounding neurons which may lead to synchronization of local neuronal activity, presumably by coexcitation of all the dendrites within the astrocyte’s domain. 8 , 18 It has been suggested that this may promote synaptic plasticity within a group of coactivated neurons. It may also play a role in generation of epileptic activity.
Activation of presynaptic neurons results in activation of astrocytes, which can release ATP. 8 , 18 ATP is converted to adenosine in the extracellular space. The increase in adenosine enhances presynaptic inhibition of transmitter release from excitatory synapses in the immediate area. Thus, astrocytic release of ATP may be important for modulating the strength of simultaneously activated synaptic connections. ATP is also one of the substances released by astrocytes that can induce surface expression of postsynaptic glutamate AMPA receptors, supporting a role in synaptic plasticity. 27
Astrocytes also synthesize and release D-serine, which binds to the glycine/serine site on the glutamate N -methyl- D -aspartic acid (NMDA) receptor. Glutamate neurotransmission via this receptor is complex in that three events must happen together. Both the glutamate and glycine binding sites on the NMDA receptor must be occupied during depolarization for the calcium influx that triggers postsynaptic actions to occur. Thus, release of D-serine by astrocytes may be essential for generation of the activity-dependent changes mediated by glutamate NMDA receptors (e.g., long-term potentiation, long-term depression), processes that are believed to underlie memory formation. 8 , 27
Recent studies indicate that astrocytes are active participants in the structural plasticity that is crucial to both the initial establishment and ongoing remodeling of connections within the nervous system. 2 , 28 It has been known for some time that dendritic spines are quite dynamic, responding to many events with rapid conformational changes (e.g., long-term potentiation is associated with increases in spine size; long-term depression is associated with decreases in spine size). Dynamic changes in astrocytes, including rapid extension and retraction of processes, have now been demonstrated utilizing time-lapse confocal microscopy ( Figure 3 ). 2 , 28 Surprisingly, the motility in astrocytes was higher than in their associated dendritic spines. This work suggests that the extent to which synapses are enveloped by glial processes may alter on a time course of minutes. Given the importance of astrocytes in maintaining the peri-synaptic environment, this has profound implications for the active role of astrocytes in modulating synaptic plasticity.
Psychiatric Conditions and Therapeutics
There is increasing evidence that alterations in glial function may be important in many conditions with neuropsychiatric symptoms, including mood disorders, schizophrenia, epilepsies, infections, toxic encephalopathies, and neurodegenerative conditions. 29 – 31
A recent review explored the evidence suggesting the participation of astrocytes in the pathophysiology of depression. 5 In brief, postmortem studies have consistently reported decreases in the number and packing density of glial cells in subjects with depression (e.g., major depressive disorder, bipolar disorder), particularly in limbic-related areas of prefrontal cortex (see Cover ). Alterations in glial cell size and shape have also been reported. Studies utilizing immunohistochemical staining for glial fibrillary acidic protein have reported profound reductions in prefrontal areas in younger subjects (<60 years of age) with depression. In contrast, neuronal changes are much less prominent in this age group. Based on these findings, it has been proposed that glial pathology precedes neuronal pathology. Animal studies suggest that stress-related increases in glucocorticoids and decreases in neurotrophic factors may contribute to this process, as excess glucocorticoids inhibit glial proliferation. 32 Astrocytes are critical for uptake of glutamate from the synaptic cleft, so decreases in this cell population might result in increased excitotoxic neuronal damage.
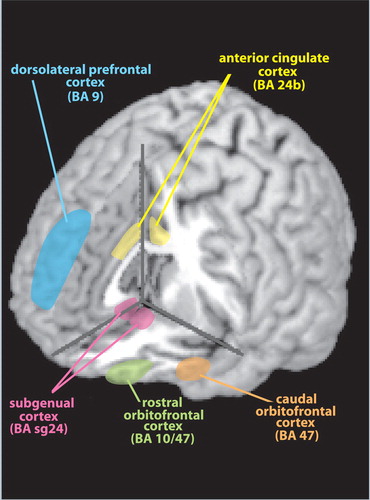
Alterations in astrocytes have also been implicated in the pathophysiology of schizophrenia. 33 Historically, glial research in schizophrenia has focused primarily on ruling out the presence of gliosis as a presumed marker for inflammatory and degenerative processes. More recently, studies have reported decreased glial cell density in several areas, although contrary findings have also been reported. 29 , 33 Decreased CSF levels of D-serine have been found in some studies, suggesting altered D-serine metabolism in schizophrenia. 34
There is growing evidence for astroglial involvement in many neurodegenerative conditions, including Alzheimer’s disease, Parkinson’s disease, Huntington’s disease, spinocerebellar ataxias, and amyotrophic lateral sclerosis. 6 , 31 , 35 A variety of mechanisms may be involved, including inflammatory and excitotoxic processes. For example, localized decreases in astrocyte glutamate transporters and uptake have been found in amyotrophic lateral sclerosis. 6 , 31 , 35 Increased levels of D-serine have also been reported. 36 Both findings support glutamate excitotoxicity as one mechanism for motor neuron degeneration.
The study of astroglia is providing insights into existing treatments as well as new avenues to explore that have great therapeutic potential. Both antidepressant medications and ECT, for example, increase expression of neurotrophic factors and glial markers. 5 , 37 A recent animal study has shown that chronic ECT results in a modest increase in S100B (secreted by astrocytes) but not of neuron-specific enolase (indicates neuronal injury) in the CSF. 38 The authors of this study noted that these results support the view that ECT does not injure the brain and suggest that ECT may act in part by enhancing protective astrocytic activity. ECT may also affect other glial populations. 5 , 39 , 40
Astrocytes produce multiple neurotrophic factors (e.g., nerve growth factor, brain-derived neurotrophic factor, glia-derived neurotrophic factor, ciliary neurotrophic factor, glia-derived nexin, epidermal growth factor, hepatocyte growth factor). 6 , 41 Many of these substances have potential therapeutic applications. Glia-derived neurotrophic factor, for example, enhances neuronal recovery from injury. 41 Since the identification of glia-derived neurotrophic factor in 1993, several other closely related agents have been discovered. This family of neurotrophic factors acts via specific receptors, raising the possibility that much smaller and more easily delivered molecules might be developed with agonist action. 41 Such agents have potential for the treatment of many disorders, including amyotrophic lateral sclerosis, Alzheimer’s disease, stroke, Parkinson’s disease, and chronic pain. The efforts to develop glia-derived neurotrophic factor as a therapeutic agent serve as an excellent example of some of the challenges.
Among other effects, glia-derived neurotrophic factor is an extremely potent protective agent for dopaminergic neurons. There has been great interest in its use in treatment of Parkinson’s disease, as recently reviewed. 41 – 43 In brief, the major difficulty is the method of delivery as glia-derived neurotrophic factor cannot cross the blood-brain barrier. Although intraventricular injection has been explored as a route of administration, diffusion of glia-derived neurotrophic factor from the ventricles into the brain substance is poor. A clinical trial utilizing this approach did not result in improved motor function, and worrisome side effects were common. Several methods of direct introduction of glia-derived neurotrophic factor to the striatum have been suggested. Localized delivery could be achieved by implantation of catheters, with automatic infusion via a pump. Such a system has a high risk of complications, but the advantage is that delivery is easily halted in the event of adverse effects. Several clinical trials have utilized this approach. Although two groups have reported beneficial effects, a randomized clinical trial was less successful. This approach is still considered promising, and basic and preclinical research continues. A different approach is to increase local synthesis of glia-derived neurotrophic factor. 42 , 43 This might be achieved by injecting genes that up-regulate synthesis of glia-derived neurotrophic factor into the target area. While this is less likely to result in surgery-related complications than an infusion pump, it would be much more difficult to reverse. A similar problem exists with the possibility of local injection of cells designed to synthesize glia-derived neurotrophic factor. Other potential problems include the danger that such cells might migrate to other brain areas, down-regulation of gene expression decreasing effectiveness, and immune rejection. An alternative is to package glia-derived neurotrophic factor-producing cells so that they cannot directly interact with the immune system and can be removed. Encapsulated cells have been successfully used for treatment of other disorders (e.g., insulin-dependent diabetes). 43 Animal studies support therapeutic effects from this approach to delivery and suggest that quite low dosages may be effective. 42
As discussed previously, astrocytes modulate neurotransmission in a variety of ways, some of which provide new therapeutic possibilities. For example, studies supporting the presence of impaired NMDA receptor functioning in schizophrenia suggest that enhancing glutaminergic neurotransmission might have therapeutic benefit. 33 , 44 , 45 The goal is to increase the excitatory influence of cortical areas into the basal ganglia. 46 The challenge is that direct administration of glutamate agonists carries a high risk of both nonspecific effects and excitotoxic damage. The multiple roles astrocytes play in modulation of glutamate neurotransmission have provided an indirect approach that is quite promising, that of enhancing occupancy of the glycine/serine binding site on the NMDA receptor. Both D-serine and glycine (full agonists) reduce symptoms when administered concurrently with antipsychotic medications. Sarcosine, which enhances the action of glycine agonists by inhibiting their reuptake into astrocytes, also has shown therapeutic benefit. These agents appear to be particularly effective in reducing the negative and cognitive symptoms of schizophrenia, symptoms that are not responsive to most typical and second generation antipsychotics. 33 , 44 , 45
CONCLUSION
Recent research has established that astroglia not only serve in supportive roles (i.e., homeostatis, structural support, and metabolism), but also play very active and dynamic roles in modulation of neuronal activity locally and regionally. Future study of astroglia may provide insight into the pathophysiology of neuropsychiatric illness and lead to new treatment interventions.
1 . Oberheim N, Wang X, Goldman S, et al: Astrocytic complexity distinguishes the human brain. Trends Neurosci 2006; 29:547–553Google Scholar
2 . Haber M, Zhou L, Murai K: Cooperative astrocyte and dendritic spine dynamics at hippocampal excitatory synapses. J Neurosci 2006; 26:8881–8891Google Scholar
3 . Nolte J: The Human Brain. St. Louis, Mosby, 2002Google Scholar
4 . Pelvig D, Pakkenberg H, Stark A, et al: Neocortical glial cell numbers in human brains. Neurobiol Aging 2007 (Epub ahead of print)Google Scholar
5 . Rajkowska G, Miguel-Hidalgo J: Gliogenesis and glial pathology in depression. CNS Neurol Disord Drug Targets 2007; 6:219–233Google Scholar
6 . Markiewicz I, Lukomska B: The role of astrocytes in the physiology and pathology of the central nervous system. Acta Neurobiol Exp (Wars) 2006; 66:343–358Google Scholar
7 . Verkhratsky A: Patching the glia reveals the functional organisation of the brain. Pflugers Arch 2006; 453:411–420Google Scholar
8 . Halassa M, Fellin T, Haydon P: The tripartite synapse: roles for gliotransmission in health and disease. Trends Mol Med 2007; 13:54–63Google Scholar
9 . Benarroch E: Neuron-astrocyte interactions: partnership for normal function and disease in the central nervous system. Mayo Clin Proc 2005; 80:1326–1338Google Scholar
10 . Ransom B, Behr T, Nedergaard M: New roles for astrocytes (stars at last). Trends Neurosci 2003; 26:520–522Google Scholar
11 . Volterra A, Meldolesi J: Astrocytes, from brain glue to communication elements: the revolution continues. Nat Rev Neurosci 2005; 6:626–640Google Scholar
12 . Nedergaard M, Ransom B, Goldman S: New roles for astrocytes: redefining the functional architecture of the brain. Trends Neurosci 2003; 26:523–530Google Scholar
13 . Giaume C, Kirchhoff F, Matute C, et al: Glia: the fulcrum of brain diseases. Cell Death Differ 2007; 14:1324–1335Google Scholar
14 . Reisin H, Colombo J: Considerations on the astroglial architecture and the columnar organization of the cerebral cortex. Cell Mol Neurobiol 2002; 22:633–644Google Scholar
15 . Colombo J, Reisin H: Interlaminar astroglia of the cerebral cortex: a marker of the primate brain. Brain Res 2004; 1006:126–131Google Scholar
16 . Korzhevskii D, Otellin V, Grigor’ev I: Glial fibrillary acidic protein in astrocytes of the human neocortex. Neurosci Behav Physiol 2005; 35:789–792Google Scholar
17 . Fellin T, Pascual O, Haydon P: Astrocytes coordinate synaptic networks, balanced excitation and inhibition. Physiology (Bethesda) 2006; 21:208–215Google Scholar
18 . Halassa M, Fellin T, Takano H, et al: Synaptic islands defined by the territory of a single astrocyte. J Neurosci 2007; 27:6473–6477Google Scholar
19 . Iadecola C, Nedergaard M: Glial regulation of the cerebral microvasculature. Nat Neurosci 2007; 10:1369–1376Google Scholar
20 . Haydon P, Carmignoto G: Astrocyte control of synaptic transmission and neurovascular coupling. Physiol Rev 2006; 86:1009–1031Google Scholar
21 . Gordon G, Mulligan S, MacVicar B: Astocyte control of the cerebrovasculature. Glia 2007; 55:1214–1221Google Scholar
22 . Newman E: New roles for astrocytes: regulation of synaptic transmission. Trends Neurosci 2003; 26:536–542Google Scholar
23 . Sykova E, Vargova L: Extrasynaptic transmission and the diffusion parameteres of the extracellular space. Neurochem Int 2008; 52:5–13Google Scholar
24 . Verkhratsky A, Kirchhoff F: Glutamate-mediated neuronal-glial transmission. J Anat 2007; 210:651–660Google Scholar
25 . Murphy S, Pearce B: Functional receptors for neurotransmitters on astroglial cells. Neuroscience 1987; 22:381–394Google Scholar
26 . Porter J, McCarthy K: Astrocytic neurotransmitter receptors in situ and in vivo. Prog Neurobiol 1997; 51:439–455Google Scholar
27 . Bains J, Oliet S: Glia: they make your memories stick! Trends Neurosci 2007; 30:417–424Google Scholar
28 . Allen N, Barres B: Signaling between glia and neurons: focus on synaptic plasticity. Curr Opin Neurobiol 2005; 15:542–548Google Scholar
29 . Cotter D, Pariante C, Everall I: Glial cell abnormalities in major psychiatric disorders: the evidence and implications. Brain Res Bull 2001; 55:585–595Google Scholar
30 . De Keyser J, Mostert J, Koch M: Dysfunctional astrocytes as key players in the pathogenesis of central nervous system disorders. J Neurol Sci 2008; 267:3–16Google Scholar
31 . Lobsiger C, Cleveland D: Glial cells as intrinsic components of non-cell-autonomous neurodegenerative disease. Nat Neurosci 2007; 10:1355–1360Google Scholar
32 . Castren E, Voikar V, Rantamaki T: Role of neurotrophic factors in depression. Curr Opin Pharmacol 2007; 7:18–21Google Scholar
33 . Kondziella D, Brenner E, Eyjolfsson E, et al: How do glial-neuronal interactions fit into current neurotransmitter hypotheses of schizophrenia? Neurochem Int 2007; 50:291–301Google Scholar
34 . Bendikov I, Nadri C, Amar S, et al: A CSF and postmortem brain study of D-serine metabolic parameters in schizophrenia. Schizophr Res 2007; 90:41–51Google Scholar
35 . Maragakis N, Rothstein J: Mechanisms of disease: astrocytes in neurodegenerative disease. Nat Clin Pract Neurol 2006; 2:679–689Google Scholar
36 . Sasabe J, Chiba T, Yamada M, et al: D-serine is a key determinant of glutamate toxicity in amyotrophic lateral sclerosis. EMBO J 2007; 26:4149–4159Google Scholar
37 . Shaltiel G, Chen G, Manji H: Neurotrophic signaling cascades in the pathophysiololgy and treatment of bipolar disorder. Curr Opin Pharmacol 2007; 7:22–26Google Scholar
38 . Busnello J, Leke R, Oses J, et al: Acute and chronic electroconvulsive shock in rats: effects on peripheral markers of neuronal injury and glial activity. Life Sci 2006; 78:3013–3017Google Scholar
39 . Wennstrom M, Hellsten J, Ekstrand J, et al: Corticosterone-induced inhibition of gliogenesis in rat hippocampus is counteracted by electroconvulsive seizures. Biol Psychiatry 2006; 59:178–186Google Scholar
40 . Ongur D, Pohlman J, Dow A, et al: Electroconvulsive seizures stimulate glial proliferation and reduce expression of Sprouty2 within the prefrontal cortex of rats. Biol Psychiatry 2007; 62:505–512Google Scholar
41 . Bespalov M, Saarma M: GDNF family receptor complexes are emerging drug targets. Trends Pharmacol Sci 2007; 28:68–74Google Scholar
42 . Lindvall O, Wahlberg L: Encapsulated cell biodelivery of GDNF: a novel clinical strategy for neuroprotection and neuroregeneration in Parkinson’s diseases? Exp Neurol 2008; 209:82–88Google Scholar
43 . Yasuhara T, Shingo T, Date I: Glial cell line-derived neurotrophic factor (GDNF) therapy for Parkinson’s disease. Acta Med Okayama 2007; 61:51–56Google Scholar
44 . Coyle J: Glutamate and schizophrenia: beyond the dopamine hypothesis. Cell Mol Neurobiol 2006; 26:365–384Google Scholar
45 . Stahl S: Novel therapeutics for schizophrenia: targeting glycine modulation of NMDA glutamate receptors. CNS Spectr 2007; 12:423–427Google Scholar
46 . Carlsson A: The neurochemical circuitry of schizophrenia. Pharmacopsychiatry 2006; 39:S10–S14Google Scholar