Neurophysiological Aspects of Synesthetic Experience
Abstract
Synesthesia is a perceptual condition in which stimulation in one sensory modality elicits a concurrent sensation in another. The authors studied possible electrophysiological correlates of synesthetic experience in 17 subjects claiming to continuously experience chromatic-graphemical synesthesia and a matched control group. Subjects had to respond to one of four numbers and one of six letters by pressing a button. Event-related potentials (ERPs) were recorded from multiple scalp sites. Most synesthetic subjects reported strong synesthetic perceptions during the experiment. The ERPs of both groups showed a distinct P300 component when subjects encountered the assigned target number or letter. Synesthetic subjects had significantly and clearly more positive waveform over frontal and prefrontal scalp regions than control subjects for target and nontarget stimuli. This electrophysiological marker is discussed in terms of cortical inhibition in synesthetic subjects and the role of prefrontal regions in multisensory integration.
Integration of information from multiple sensory channels is a necessary prerequisite for many tasks with which the nervous system is entrusted. Most notably, the visual and vestibular systems are deeply intertwined so as to induce the sensation of movement when the vestibular system is activated. Also, intersensory bias can occur in a number of instances—for example, vision can influence perception of auditory or proprioceptive input and vice versa.1 Most notably, this effect has been exploited by ventriloquists who fool the audience about the source of their dummy's voice.2
Besides these common examples of intersensory integration, there is a rare phenomenon termed synesthesia, in which a commingling of sensory experiences from different modalities occurs in otherwise neurologically and psychologically healthy individuals. For example, those individuals might experience the intense feeling of touching shapes upon tasting something (taste–shape synesthesia) or they might see colors upon hearing words or sounds (colored hearing synesthesia). Synesthetic phenomena can also be found in normal individuals, albeit to a much lesser degree—for instance, some people have intense somesthetic sensations upon hearing music.3,4
Several pieces of evidence support the notion that indeed synesthetic experience has a neural basis:
1. | There is a remarkable consistency of associations (e.g., sound–color associations) over time.5,6 For example, Baron-Cohen et al.5 found a consistency of 92% of color–sound associations after 1 year in 13 synesthetic subjects but only a 37% consistency (after 1 week) in a control group. | ||||
2. | There appears to be a strong familial clustering of synesthesia, with a female-to-male ratio of 6:1. This has been interpreted as reflecting a genetic basis for synesthesia.7 | ||||
3. | There is evidence that synesthesia can be acquired in the course of neurological illnesses such as multiple sclerosis, temporal arteritis, tumors to the sella region, and others.8 | ||||
4. | Synesthetic experiences can be induced by ingestion of drugs such as mescaline.9,10 | ||||
5. | There appear to be differences between nonsynesthetes and synesthetes in measures of cerebral blood flow.11 |
With regard to possible mechanisms through which brain circuits might subserve synesthetic experience, a wealth of data has accumulated showing the presence of multisensory neurons at several levels of the central nervous system.12 However, although a number of studies address the phenomenology of synesthetic experience6,7,9,13–17 experiments trying to pinpoint the neural basis of synesthesia have been scarce. Cytowic and Wood18 have used the nontomographic xenon-133 method to assess cerebral blood flow in a single subject with taste–shape synesthesia. They concluded that the sensory integration leading to the synesthetic sensation takes place in the limbic system (a region not actually assessed in their study) and that neocortical structures appeared to be inhibited. In a more recent study using the superior PET activation technique in 6 synesthetic (colored hearing) and 6 control subjects, Paulesu et al.11 found activation in visual association areas (posterior inferior temporal cortex, parieto-occipital junction) as well as activation of right prefrontal cortex, insula, and superior temporal gyrus. Notably, primary visual areas did not show blood flow changes during activation.
In the present study we sought to investigate the neurophysiology of synesthesia by the recording of event-related brain potentials (ERPs). These are small voltage fluctuations occurring in response to a stimulus or a cognitive event and can be extracted from the ongoing EEG by averaging techniques.19 The main advantages of ERPs are their high temporal resolution, noninvasive nature, and repeatability. Their applications in neurology and psychology have been manifold and include the investigation of visual perception,20,21 memory,22 and language processes.23 A study by Hillyard et al.24 had subjects attend to visual or auditory stimuli at a particular location and examined the effects on the ERPs to stimuli of the unattended (visual or auditory) modality. It was found that ERPs to stimuli of the unattended modality were enhanced in amplitude at the attended location, suggesting a mapping of all stimuli onto a common auditory-visual space. These results attest to the utility of ERPs in the investigation of sensory processing and suggest a preferred allocation of resources to stimuli at the attended location, regardless of modality.
For the current study we adapted the well-known oddball paradigm,25 which requires the subjects to respond to a rare target stimulus in a series of distractor stimuli. Subjects were confronted with stimuli (numbers and letters) that were known to elicit synesthetic experiences in the selected synesthetes. We hypothesized that differences in the processing of the stimuli between synesthetic and control subjects would show up in the ERPs. Specifically, in light of the PET data presented by Paulesu et al.11 on synesthetic subjects and single-cell data from primates and other species indicating that the frontal and prefrontal areas are richly endowed with multisensory neurons,26–30 we predicted differences between synesthetic and control subjects over frontal regions.
METHODS
Subjects
Seventeen synesthetic (SYN) subjects (14 women, 3 men; age range 20–52 years, mean 32.4) were selected from a much larger sample of more than a hundred persons claiming to have synesthetic experiences. The original sample was collected after a feature article on synesthesia had appeared in a popular journal. Those subjects were carefully screened for psychiatric, neurological, and ophthalmological diseases by one of the authors (K.T.) and were found to be healthy. The subjects were selected for color-graphemic synesthesia. The original sample also included subjects with colored hearing, colored music, and more complex synesthesias. Thus, all SYN subjects reported continuous synesthetic perceptions when they read letters and/or numbers. Nine subjects reported that the perceived color depended on the vowels that a given word contains, 6 said it depended on both the vowels and the consonants, and 2 reported that the first letter exclusively determined the color. The perceptions were described as unstructured patches or blobs of color. Subjects in which two or more letters determined the synesthesia reported several patches of different colors next to each other rather than one patch of a mixed color. All subjects reported that the synesthesias “automatically” accompanied reading and that they made no efforts to elicit the synesthetic experiences.
Eleven SYN subjects had obtained a university diploma, 4 were expert workers, and 2 were homemakers. Four of the female subjects reported at least one other case of synesthesia in their families. None of the male subjects had any relatives with known synesthesia. Most SYN subjects dated their synesthesias back to the preschool age (latest onset 10 years).
A group of age- and sex-matched control (CON) subjects was obtained in which no subject had ever experienced any synesthesia.
All subjects were right-handed and gave their informed consent to participate. The study protocol was approved by the local ethics committee.
Stimuli
Only visual stimuli were used in the present study. In Experiment 1 the subjects viewed a sequence of letters appearing in white against a dark background in the middle of a video monitor. The viewing distance was 80 cm, and height (width) of stimuli was 4 (3) cm. The duration of the letters was 300 ms, with an interstimulus interval varying randomly between 1,500 and 2,500 ms. Before each experimental run, subjects were informed as to which of the letters would serve as a target during that particular run and had to be responded to by pressing a button held in the right hand. Each run contained 90 stimuli, and 6 runs were administered to each subject. Fifteen letters in each run served as targets, and the others served as distractors. The letters A, E, I, O, U, and M were used as distractors and targets.
The mode of presentation, timing, and task of Experiment 2 was very similar to Experiment 1 except that the numbers 1, 4, 5, and 18 served as distractors and targets.
Recording and Data Quantification
The EEG was recorded from 29 scalp sites, including all standard sites of the international 10-20 system, by using tin electrodes mounted in an elastic cap (Electro-Cap, Inc., Eaton, OH). The reference electrode was placed on the right mastoid process, and additional electrodes were used to monitor eye movements for artifact rejection purposes. Biosignals were amplified (low-pass filter settings 100 Hz, time constants 10 seconds), digitized with a 4 ms resolution, and stored for later offline analysis. ERPs were averaged according to the different stimulus categories (e.g., targets vs. nontargets) for 1,024-ms epochs, starting 100 ms before stimulus onset.
The resulting waveforms were quantified by using mean amplitude measures relative to the mean amplitude of the 100-ms baseline period preceding the stimulus. The amplitude measures were entered in a repeated-measures analysis of variance with group (SYN vs. CON) as between-subjects factor and stimulus (target vs. nontarget) and electrode site as within-subjects factors. Two sets of analyses were conducted, one on the three midline electrodes (Fz, Cz, and Pz), which were entered as levels for the electrode site factor, and a second set on the parasagittal electrodes (Fp1/Fp2, F3/F4, C3/C4, P3/P4, O1/O2), with data arranged to yield a hemisphere factor (levels left, right) and an electrode site factor (five levels from anterior to posterior). The Greenhouse-Geisser correction31 for inhomogeneity of covariance was used whenever applicable, and reported P-values are corrected.
RESULTS
Subjective Reports of the Synesthetic Subjects
Fourteen subjects reported definite synesthesia throughout the experiment, 2 were not sure, and 1 subject denied synesthesia during the experiment.
Twelve SYN subjects reported constant appearance of colors with every character they saw, whereas 2 had stronger perceptions with the vowels. Of the latter 2 subjects, 1 did not experience synesthesia when seeing the “M.”. The same 2 persons reported seeing the “18” as a patch-pattern of the colors for the single digits, standing side to side. All subjects felt that synesthetic perception was unrelated to the target status of a letter.
Behavioral Data
The mean reaction time to target stimuli in the letter task (Experiment 1) was 480 ms (SD=97) for SYN and 500 ms (SD=116) for CON subjects, which was not a significant difference. Similarly, hit rates did not significantly differ between the two subject groups (SYN 95.2%, SD=4.2; CON 94.5%, SD=4.5).
Analogous results were obtained in the number task (Experiment 2), in which reaction times were 460 ms (SD=102) for SYN and 484 ms (SD=134) for CON (not significant). The percentage of correct responses was 95.7% (SD=4.1) in the SYN and 92% (SD=5.1) in the CON subjects (not significant).
Event-related Potentials
Experiment 1:
The grand average potentials (n=17) for the SYN and CON groups are shown in Figure 1A and Figure 2A. Concerning the difference between target and nontarget stimuli, a large-amplitude P300 component25 with a parietal maximum was observed for the targets with an approximate peak latency of 430 ms and a parietal maximum in both groups. Figure 1A shows the grand average potentials for the electrode site Pz, where the P300 component had its maximum amplitude. Regarding the critical comparison of this study, the waveforms for SYN and CON subjects were virtually superimposable at the parietal site for both stimulus classes. A clear-cut difference emerged, however, over frontal and central sites, where the waveforms of the CON group took a more negative course beginning approximately 150 ms after stimulus onset (Figure 2A). Figure 3 illustrates the interindividual variability in the two groups. Although the variability in the two groups appears to be similar, the synesthetic subjects as a group have more positive ERPs at the frontal sites. This pronounced difference in distribution of ERPs between the two groups is best illustrated by looking at mean voltage amplitudes, in the time window 300–600 ms poststimulus, of electrodes in two parasagittal lines and two temporal lines (Figure 4A). The voltages at parieto-occipital sites are similar for the two groups, whereas marked differences are apparent over frontal areas.
The statistical results are summarized in Table 1. For both midline and parasagittal sites, highly significant effects were obtained for the stimulus factor for most time windows. A main effect of the factor group was found in only a single time window. The highly different scalp distributions of the ERPs between the groups was reflected in group by electrode-site interaction effects.
Experiment 2:
The results from the number task are presented in an analogous fashion. Figure 1B shows the very similar potentials at the parietal site in the two groups for every stimulus class displaying the expected difference between target and nontarget stimuli. Figure 2B shows the ERPs from midline locations, with a pronounced difference between groups over frontal and central sites and no clear-cut differences at the parietal site. The corresponding mean voltage amplitudes are shown in Figure 4B. Again, marked differences in distribution of waveforms were found between groups for both stimulus classes. The differences were found predominantly over anterior scalp regions.
The statistical results are summarized in Table 1. Again, the greater positivity to the targets was reflected in a main effect of the stimulus factor in several time windows. A main effect of group was found for only the 200–300-ms time window, whereas group by electrode-site interactions reflecting the different distributions between the two groups were found for most of the time windows.
DISCUSSION
The aim of the current study was to screen for electrophysiological differences between subjects experiencing synesthesia and matched control subjects. In two experiments, highly significant differences emerged in the distribution of brain activity, the waveforms of synesthetic subjects being more positive over anterior scalp areas. On the other hand, task-related ERP changes, such as the late positive component indexing the evaluation of the target stimulus, appeared by and large the same between the two groups, and behavioral indices of task performance did not differ between the two groups.
In order to explore the possible links of these results with previous data, we will structure the discussion in the following way: first, we will address their relation to other findings from the ERP literature; then, we will turn to the question of how they relate to data from the synesthesia literature, especially functional imaging data; and finally, we will present a tentative explanation of the data to be tested in further studies.
Relationship to ERP Data
Slow ERPs have been used increasingly to monitor the brain's responses in a number of tasks. Slow brain potentials occur on a time scale ranging from several hundred milliseconds up to several seconds. Slow negative potentials have been observed in a wide variety of applications, such as selective attention,32 mental arithmetic,33 mental rotation,34 and concept formation,35 to name but a few. A prevailing view of long-duration slow potentials has been that the surface negative potentials reflect an activation of the underlying cortical areas,36,37 whereas positivities are believed to reflect cortical inhibition. This view is based on the finding that in a variety of animal models, the columnar cortical architecture and the resulting orientation of the neurons cause excitatory postsynaptic potentials in the upper cortical layers to result in surface negativities.38,39
Applying this reasoning to the current set of data, one is led to conclude that the increased positivity overlying anterior brain areas in the synesthetic group reflects an inhibition of frontal and prefrontal structures. We will return to this possibility later in this discussion.
In interpreting ERP results one should bear in mind that event-related potentials in general reflect cortical activity, with little contribution of subcortical structures to the field observed at the scalp. One reason for this is that subcortical structures such as the basal ganglia have an architecture that would lead to a closed field; hence there is no orderly orientation of the neurons, leading to a cancellation of the fields of the single neurons. Thus, any influence of subcortical structures in the generation or suppression of synesthetic experience can be assessed only indirectly, via its influences on cortical potentials.
Relationship to Other Results on Synesthesia
The present data bear some resemblance to the PET imaging study by Paulesu et al.11 Besides activation of a number of higher order visual association areas (posterior inferior temporal cortex, parieto-occipital junction), these authors demonstrated activation in extravisual areas as well, such as the right prefrontal cortex, the insula, and the superior temporal gyrus. The frontal activation was interpreted by these authors as “due to the attentional demands generated by the perceptual demands of hearing words, which in this case convey double and sometimes conflicting information.” They also refer to work by Zeki et al.,40 who were able to show with the stimulation PET method that illusory visual motion perception led to a prefrontal activation explained by the attentional demands carried by that task.
Role of Prefrontal Cortex
In interpreting our data, we must consider the functions of prefrontal cortex. Distractibility is one of the key features observed in animals or humans with lesions to the prefrontal cortex,41–43 and it is explained by a failure to suppress attention to irrelevant stimuli. The normal prefrontal output results in an inhibitory modulation of subcortical and cortical structures.44,45
The question remains of how to interpret the frontal positivity in synesthetic subjects in the present study. Following the reasoning of Elbert and Rockstroh,36 this frontal positivity would have to be interpreted as an inhibition of the frontal lobe, which in turn should lead to an increased distractibility and possibly to a leakage between the modalities. An alternative interpretation draws on the notion that frontal areas are richly endowed with multisensory neurons and that this area could serve as the anatomical site in which synesthesia occurs.26–30 The inhibition occurring over anterior brain regions could be thought of as an effort of the nervous system to keep the synesthetic perceptions to a minimum, since these are interfering with normal perception and are leading to sensory conflicts. The predictions generated by these alternative theories are currently being tested in our laboratory.
ACKNOWLEDGMENTS
The authors are grateful to the subjects for their participation. Jobst Kilian provided excellent technical support. K.S. was supported by a fellowship of the Biomedical Exchange Program between Germany and the United States. T.F.M. was supported by a grant of the Hermann and Lilly Schilling Foundation (TS 013/177/96). Thanks to Marta Kutas for providing a stimulating work environment during the writing of this article.
![]() |
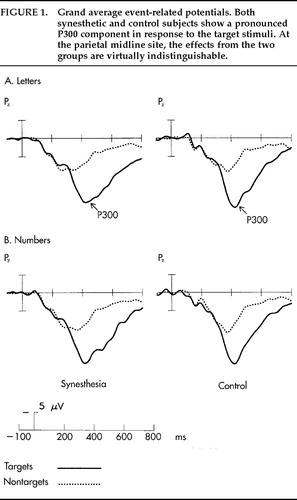
FIGURE 1. Grand average event-related potentials. Both synesthetic and control subjects show a pronounced P300 component in response to the target stimuli. At the parietal midline site, the effects from the two groups are virtually indistinguishable.
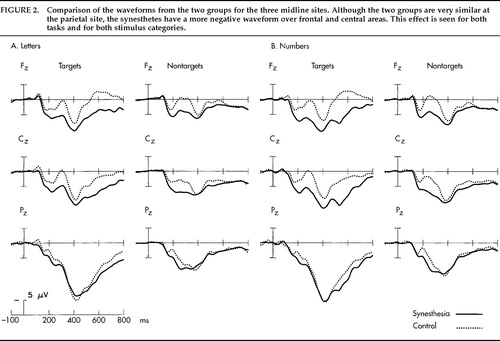
FIGURE 2. Comparison of the waveforms from the two groups for the three midline sites. Although the two groups are very similar at the parietal site, the synesthetes have a more negative waveform over frontal and central areas. This effect is seen for both tasks and for both stimulus categories.
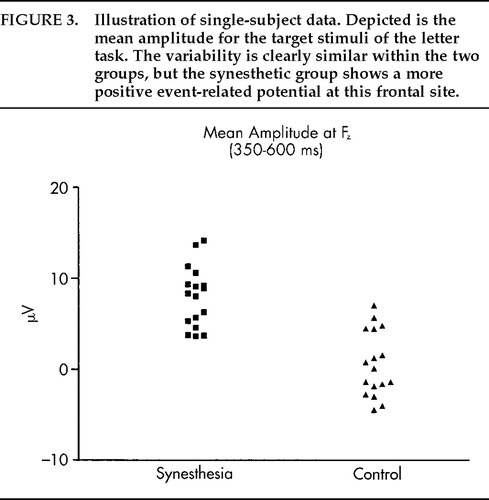
FIGURE 3. Illustration of single-subject data. Depicted is the mean amplitude for the target stimuli of the letter task. The variability is clearly similar within the two groups, but the synesthetic group shows a more positive event-related potential at this frontal site.
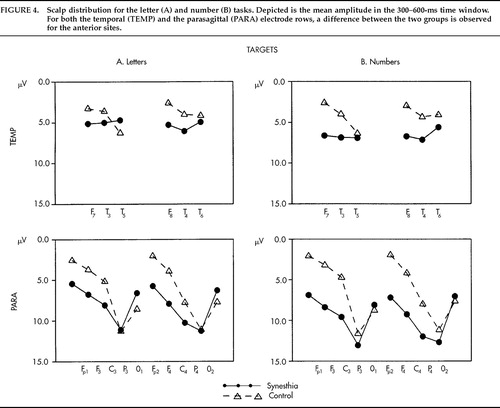
FIGURE 4. Scalp distribution for the letter (A) and number (B) tasks. Depicted is the mean amplitude in the 300–600-ms time window. For both the temporal (TEMP) and the parasagittal (PARA) electrode rows, a difference between the two groups is observed for the anterior sites.
1 Welch RB, Warren DH: Intersensory interactions, in Handbook of Perception and Human Performance, vol 1: Sensory Processes and Perception, edited by Boff KR, Kaufman L, Thomas JP. New York, Wiley, 1986, chap 25, pp 1–36Google Scholar
2 Howard IP, Templeton WB: Human Spatial Orientation. London and New York, Wiley, 1966Google Scholar
3 Critchley M: Ecstatic and synesthetic experience during musical perception, in Music and Brain: Studies in the Neurology of Music, edited by Critchley M, Henson RA. Springfield, IL, Charles C Thomas, 1977, pp 187–205Google Scholar
4 Henson RA: Neurological aspects of musical experience, in Music and Brain: Studies in the Neurology of Music, edited by Critchley M, Henson RA. Springfield, IL, Charles C Thomas, 1977, pp 387–401Google Scholar
5 Baron-Cohen S, Harrison J, Goldstein LH, et al: Coloured speech perception: is synaesthesia what happens when modularity breaks down? Perception 1993; 22:419–426Google Scholar
6 Svartdal F, Iversen T: Consistency in synesthetic experience to vowels and consonants. Scand J Psychol 1989; 30:220–227Crossref, Medline, Google Scholar
7 Baron-Cohen S, Burt L, Smith-Laittan F, et al: Synaesthesia: prevalence and familiality. Perception 1996; 25:1073–1079Crossref, Medline, Google Scholar
8 Jacobs L, Karpik A, Bozian D, et al: Auditory-visual synesthesia: sound induced photisms. Arch Neurol 1981; 38:211–216Crossref, Medline, Google Scholar
9 Cytowic RE: Synesthesia: A Union of the Senses. New York, Springer, 1989Google Scholar
10 Simpson L, McKellar P: Types of synaesthesia. J Ment Sci 1955; 101:141–147Crossref, Medline, Google Scholar
11 Paulesu E, Harrison J, Baron-Cohen S, et al: The physiology of coloured hearing: a PET activation study of colour-word synaesthesia. Brain 1995; 118:661–676Crossref, Medline, Google Scholar
12 Stein BE, Meredith MA: The Merging of the Senses. Cambridge, MA, Bradford Books, 1993Google Scholar
13 Baron-Cohen S, Wyke MA, Binnie C: Hearing words and seeing colours: an experimental investigation of synaesthesia. Perception 1987; 16:761–767Crossref, Medline, Google Scholar
14 Glicksohn J, Salinger O, Roychman A: An exploratory study of syncretic experience: eidetics, synaesthesia and absorption. Perception 1992; 21:637–642Crossref, Medline, Google Scholar
15 Marks LE: On colored hearing synesthesia: cross-modal translations of sensory dimensions. Psychol Bull 1975; 82:303–331Crossref, Medline, Google Scholar
16 Marks LE: The Unity of the Senses: Interrelations Among the Modalities. New York, Academic Press, 1978Google Scholar
17 Rader CM, Tellegen A: An investigation of synesthesia. J Pers Soc Psychol 1987; 52:981–987Crossref, Google Scholar
18 Cytowic RE, Wood FB: Synesthesia, II: psychophysical relations in the synesthesia of geometrically shaped taste and colored hearing. Brain Cogn 1982; 1:36–49Crossref, Medline, Google Scholar
19 Hillyard SA, Picton TW: Electrophysiology of cognition, in Handbook of Physiology, sect 1: Neurophysiology, edited by Plum F. New York, American Physiological Society, 1987Google Scholar
20 Hillyard SA, Münte TF: Selective attention to color and locational cues: an analysis with event-related brain potentials. Perception and Psychophysics 1984; 36:185–198Crossref, Medline, Google Scholar
21 Johannes S, Münte TF, Heinze HJ, et al: Luminance and spatial attention effects on early visual processing. Brain Res Cogn Brain Res 1995; 2:189–205Crossref, Medline, Google Scholar
22 Paller KA, Kutas M: Brain potentials during memory retrieval provide neurophysiological support for the distinction between conscious recollection and priming. J Cogn Neurosci 1992; 4:375–391Crossref, Medline, Google Scholar
23 Kutas M, Van Petten C: Event related brain potential studies of language, in Advances in Psychophysiology 3, edited by Ackles PK, Jennings JR, Coles MGH. Greenwich, CT, JAI Press, 1988, pp 380–412Google Scholar
24 Hillyard SA, Simpson GV, Woods DL, et al: Event-related brain potentials and selective attention to different modalities, in Cortical Integration, edited by Reinoso-Suarez F, Ajmone-Marsan C. New York, Raven, 1984, pp 395–414Google Scholar
25 Polich J: P300 in clinical applications: meaning, method, and measurement. American Journal of EEG Technology 1991; 31:201–231Crossref, Google Scholar
26 Rizzolatti G, Scandolara C, Matelli M, et al: Afferent properties of periarcuate neurons in macaque monkeys, I: somatosensory responses. Behav Brain Res 1981; 2:125–146Crossref, Medline, Google Scholar
27 Rizzolatti G, Scandolara C, Matelli M, et al: Afferent properties of periarcuate neurons in macaque monkeys, II: visual responses. Behav Brain Res 1981; 2:147–163Crossref, Medline, Google Scholar
28 Ito SI: Prefrontal unit activity of macaque monkeys during auditory and visual reaction time tasks. Brain Res 1982; 247:39–47Crossref, Medline, Google Scholar
29 Clemo HR, Stein BE: Organization of a fourth somatosensory area of cortex in cat. J Neurophysiol 1983; 50:910–925Crossref, Medline, Google Scholar
30 Benevento LA, Fallon JH, Davis B, et al: Auditory visual interaction in single cells in the cortex of the superior temporal sulcus and the orbital frontal cortex of macaque monkey. Exp Neurol 1977; 57:849–872Crossref, Medline, Google Scholar
31 Jennings JR, Wood CC: The epsilon-adjustment procedure for repeated analyses of variance. Psychophysiology 1976; 13:277–278Crossref, Medline, Google Scholar
32 Hansen JC, Hillyard SA: Selective attention to multidimensional auditory stimuli. J Exp Psychol: Hum Percept Perform 1983; 9:1–19Crossref, Medline, Google Scholar
33 Rösler F, Heil M: Towards a functional categorization of slow waves: taking into account past or future events? Psychophysiology 1991; 28:344–358Google Scholar
34 Farah MJ, Peronnet F: Event-related potentials in the study of mental imagery. J Psychophysiol 1989; 3:99–109Google Scholar
35 Lang M, Lang W, Uhl F, et al: Slow negative potential shifts indicate verbal cognitive learning in a concept formation task. Human Neurobiology 1987; 6:183–190Medline, Google Scholar
36 Elbert T, Rockstroh B: Threshold regulation: a key to the understanding of the combined dynamics of EEG and event-related potentials. J Psychophysiol 1987; 4:317–333Google Scholar
37 Birbaumer N, Elbert T, Canavan AGM, et al: Slow potentials of the cerebral cortex and behaviour. Physiol Rev 1990; 70:1–41Crossref, Medline, Google Scholar
38 Creutzfeldt OD: Cortex Cerebri: Leistung, strukturelle und funktionelle Organisation der Hirnrinde [Cerebral cortex: performance, structural and functional organization of the brain]. Berlin, Springer-Verlag, 1972Google Scholar
39 Speckmann EJ, Caspers H, Elger CE: Neuronal mechanisms underlying the generation of field potentials, in Self-Regulation of the Brain and Behavior, edited by Elbert T, Rockstroh B, Lutzenberger W, et al. Berlin, Springer, 1984, pp 9–25Google Scholar
40 Zeki S, Watson JDG, Frackowiak RSJ: Going beyond the information given: the relation of illusory visual motion to brain activity. Proc R Soc Lond Biol 1993; 252:215–222Crossref, Medline, Google Scholar
41 Bartus RT, Levere TE: Frontal decortication in rhesus monkeys: a test of the interference hypothesis. Brain Res 1977; 119:233–248Crossref, Medline, Google Scholar
42 Fuster JM: The Prefrontal Cortex. New York, Raven, 1980Google Scholar
43 Milner B: Some cognitive effects of frontal lesions in man. Phil Trans R Soc Lond 1982; 298:211–226Crossref, Medline, Google Scholar
44 Alexander GE, Newman JD, Symmes D: Convergence of prefrontal and acoustic inputs upon neurons in the superior temporal gyrus of the awake squirrel monkey. Brain Res 1976; 116:334–338Crossref, Medline, Google Scholar
45 Edinger HM, Siegel A, Troiano R: Effect of stimulation of prefrontal cortex and amygdala on diencephalic neurons. Brain Res 1975; 97:17–31Crossref, Medline, Google Scholar