Comparison of T1Rho MRI, Glucose Metabolism, and Amyloid Burden Across the Cognitive Spectrum: A Pilot Study
Abstract
Objective:
The pathological cascades associated with the development of Alzheimer’s disease (AD) have a common element: acidosis. T1rho MRI is a pH-sensitive measure, with higher values associated with greater neuropathological burden. The authors investigated the relationship between T1rho imaging and AD-associated pathologies as determined by available diagnostic imaging techniques.
Methods:
Twenty-seven participants (men, N=13, women, N=14; ages 55–90) across the cognitive spectrum (healthy control subjects [HCs] with normal cognition, N=17; participants with mild cognitive impairment [MCI], N=7; participants with mild AD, N=3) underwent neuropsychological testing, MRI (T1-weighted and T1rho [spin-lattice relaxation time in the rotating frame]), and positron emission tomography imaging ([11C]Pittsburg compound B for amyloid burden [N=26] and [18F]fluorodeoxyglucose for cerebral glucose metabolism [N=12]). The relationships between global T1rho values and neuropsychological, demographic, and imaging measures were explored.
Results:
Global mean and median T1rho were positively associated with age. After controlling for age, higher global T1rho was associated with poorer cognitive function, poorer memory function (immediate and delayed memory scores), higher amyloid burden, and more abnormal cerebral glucose metabolism. Regional T1rho values, when controlling for age, significantly differed between HCs and participants with MCI or AD in select frontal, cingulate, and parietal regions.
Conclusions:
Higher T1rho values were associated with greater cognitive impairment and pathological burden. T1rho, a biomarker that varies according to a feature common to each cascade rather than one that is unique to a particular pathology, has the potential to serve as a metric of neuropathology, theoretically providing a measure for assessing pathological status and for monitoring the neurodegeneration trajectory.
The pathophysiology of Alzheimer’s disease (AD) has been characterized as multifactorial processes that lead to the death of cholinergic neurons and eventually the clinical symptoms of AD. Humpel (1) proposed a “common unifying hypothesis” that combines the vasculopathy (i.e., impaired blood flow) and β-amyloid cascade hypotheses to explain how amyloid pathology, tau pathology, vascular insufficiency, metabolic disturbances, and oxidative stress all—jointly or individually—can lead to cell death. An alternative explanation is posed by the mitochondrial cascade hypothesis (2). This hypothesis proposes that mitochondrial function declines until bioenergetic failure occurs. This failure precipitates β-amyloid production and subsequent plaque deposition, as well as tau phosphorylation, leading to tangle formation and synaptic loss with subsequent neurodegeneration (3).
A common element in each of these pathological cascade hypotheses is the presence of acidosis. Acidosis is the result of increased levels of CO2 or accumulation of acidic byproducts of dysfunctional metabolism. Acidosis may represent an early sign of mitochondrial dysfunction and may also be a facilitator of the pathological process itself by enhancing β-amyloid aggregation, production of reactive oxygen species (4), and cholinergic cell death (1).
Brain imaging techniques are currently available for the assessment of many of the branches of pathological cascade, including vascular insufficiency (cerebral blood flow imaging [15O]water], arterial spin labeling), glucose metabolic disturbances ([18F]fluorodeoxyglucose [FDG]), β-amyloid clearance and plaques (amyloid imaging agents, e.g., [18F]florbetapir, [18F]florbetaben, [18F]flutemetamol, and [11C]Pittsburgh Compound B [PiB], tau pathology (e.g., [18F]flortaucipir), and cell death with associated atrophy (structural MRI). However, each technique examines a specific component of AD-associated pathology in isolation. None of these techniques supplies a composite picture of the status of the individual on the dementia pathological trajectory; however, each pathology contributes to cognitive deterioration. Imaging measures that vary with the overall burden or that assess a common feature (e.g., acidosis) of pathologies that affect neuropsychological function may represent a means of compositing all of these pathological processes into a single metric.
Several previous studies demonstrated that T1rho MRI (spin-lattice relaxation in the rotating frame) is sensitive to pH (5–10). A consistent finding in these studies was that higher T1rho relaxation times corresponded to a lower pH (higher T1rho=lower pH=more acidic). This relationship held across a wide range of magnetic field strengths (3.0–9.4 T) and spin-lock amplitudes (2.9–94 μT). In addition, Owusu et al. (11) found that the strongest contribution to changes appears to be pH at 3-T using spin-lock amplitudes that can be achieved with U.S. Food and Drug Administration limits of subject heating. Other studies (7, 12–14) have evaluated the use of T1rho MRI to examine brain pH, and some have explored the potential utility of this technique in distinguishing the pathologies underlying panic disorder (15), bipolar disorder (16, 17), and premanifest Huntington’s disease (18). In each disorder, the T1rho signal was elevated in specific regions known to be adversely affected in patients with the particular neuropsychiatric disorder compared with control subjects (e.g., the striatum in Huntington’s disease [18]).
Studies on AD have revealed differences in the T1rho signal that may distinguish healthy control subjects (HCs) from individuals with cognitive impairment (mild cognitive impairment [MCI] and AD) (19–23). The T1rho signal in the temporal lobe alone (20, 22) and in combination with CSF biomarkers (23) was shown to distinguish study groups. In addition, the T1rho signal in the hippocampus (21) was potentially useful in distinguishing AD from Parkinson’s disease (PD) without (24) and with associated dementia (PDD) (21, 25). Also, the T1rho signal in the substantia nigra was correlated with the asymmetry of PD motor symptoms (Unified Parkinson’s Disease Rating Scale scores) (25).
The AD-related research with T1rho has focused on medial temporal lobe structures and, except for the work conducted by Haris et al. (23), has depended solely on subject classification based on neuropsychological or clinical evaluations. None of the dementia-related investigations examined the T1rho signal, both globally and regionally, compared with imaging-based measures of the AD-associated pathological processes (e.g., amyloid and glucose hypometabolism).
The purpose of the present study was to investigate the relationship between T1rho imaging and AD-associated pathologies as determined by available diagnostic imaging techniques. The techniques applied utilized measures of brain metabolism, specifically glucose metabolism with FDG, and AD-associated pathology, specifically amyloid burden with [11C]PiB positron emission tomography (PET) imaging. It was hypothesized that higher T1rho signal would be associated with more abnormal function or greater pathological burden, specifically lower FDG uptake, greater AD-associated pathology (i.e., amyloid burden), and poorer cognitive performance. Age was considered as a potentially important covariate in the analyses because of the conflicting findings regarding the association between T1rho and age. Zhao et al. (26) observed significant increases in T1rho signal associated with age-related changes in rat brain. These findings are consistent with those of Menyhárt et al. (27), who found tissue acidosis in aged rat brain due to alterations in pH shift induced by spreading depolarization. It was hypothesized that the spreading depolarization-induced acidic pH shift was the result of a persistent elevation in the lactate concentration due to reductions in the facilitated diffusion of lactate into the bloodstream in aged brain.
In human studies, some authors have found no relationship between T1rho and age (21–23) or nonstatistically significant increases in the T1rho signal and age (20) when examining limited areas of the brain (e.g., the medial temporal lobe and hippocampus). However, Watts et al. (28) found significant decreases with age in cortical gray matter and in the caudate, putamen, hippocampus, amygdala, and nucleus accumbens but significant increases with age in white matter tracts. Recent in vitro studies of human neocortical tissues resected from patients who had epilepsy surgery revealed a mild acidification with age (29). It was hypothesized that the age-related decrease in neuronal intracellular pH may have been associated with beneficial neuroprotection by limiting excitotoxicity. On the other hand, intracellular acidification can induce apoptosis and promote the development of Alzheimer’s dementia (30). Therefore, age was considered in all analyses.
Methods
Participants
Male and postmenopausal female participants, ages 55–90, who were cognitively healthy and had MCI or early AD based on clinical criteria were eligible for inclusion. Participants were excluded if they were unable to undergo MRI or if they had a history of or current diagnosis of major psychiatric disorders (other than dementia), a history of abuse of psychoactive substances or medications, a history of stroke, a history of head injury with loss of consciousness >30 minutes, or a history of other neurological disorders or systemic illness that could potentially affect cognition or brain function (other than a diagnosis of AD or MCI) or that could affect their safety or comfort while undergoing imaging studies. The study was approved by the University of Iowa Institutional Review Board and Medical Radiation Protection Committee, and written informed consent was obtained from all participants. The participants were placed into cognitive classifications on the basis of methods validated by the Alzheimer’s Disease Neuroimaging Initiative (31). If a participant had already undergone a comprehensive clinical evaluation with confirmation of the diagnosis through longitudinal follow-up, then his or her current clinical diagnosis was used.
PET Radiopharmaceuticals
[11C]PiB was prepared according to the methods and met all specifications detailed in the chemistry, manufacturing, and controls section of the University of Iowa Investigational New Drug Application (32). FDG was produced in accordance with criteria established by the University of Iowa Abbreviated New Drug Application for [18F]fluorodeoxyglucose Injection.
Imaging
All participants underwent MRI, including T1rho imaging, neuropsychological testing, and [11C]PiB PET imaging. A subset of participants also underwent FDG imaging for assessment of cerebral glucose metabolism. All PET imaging was performed on a Siemens ECAT EXACT HR+ with transmission imaging ([68Ge]) for attenuation correction performed prior to the injection of the radiotracers. MRI was performed on a 3.0-T Siemens TIM Trio MRI Scanner. All image analyses were performed with the PMOD suite of tools (PVIEW, PFUSION, PNEURO, PXMOD, PKIN, and PALZ, versions 3.7 and 3.8, PMOD Technologies, Zurich.).
FDG
Participants fasted for a minimum of 6 hours before FDG administration. Blood glucose levels were measured before FDG administration, and for all participants, the levels were ≤200 mg/dL (range, 70–188 mg/dL). FDG (185 MBq±10% [5 mCi±10%] intravenous) was administered and imaged dynamically for 60 minutes. The scanner was in a quiet, darkened room, and participants had their eyes open and ears unplugged during the uptake and imaging period. Dynamic data were iteratively reconstructed (Gaussian 3.0, six iterations/16 subsets, zoom=2.6, attenuation, scatter, decay and deadtime correction=ON) and summed from 30 to 60 minutes.
[11C]PiB Imaging
Participants were injected with [11C]PiB (555 MBq±10% [15 mCi±10%] intravenous) on the scanner and imaged dynamically for 90 minutes. The ambient conditions during imaging were similar to those experienced during the FDG condition. Dynamic data were iteratively reconstructed (Gaussian 3.0, six iterations/16 subsets, zoom=2.6, attenuation, scatter, decay and deadtime correction=ON), and a summed image was created with the data from 50 to 70 minutes postadministration.
MRI
A multimodal MRI protocol was collected that included three-dimensional high-resolution anatomical images (T1-three-dimensional magnetization-prepared rapid gradient-echo and T2-three-dimensional variable flip angle spin-echo sequences) and volumetric T1rho images (three-dimensional fast low-angle shot: TE=3.5 ms, TR=7.6 ms, flip angle=15°, field of view=240×240×100, matrix=128×128×20, bandwidth=260 Hz/pixel, B1 amplitude=250 Hz, spin-lock times=10, 60 ms) (33). The T1rho data were conducted during rest in order to parallel the [11C]PiB and FDG conditions.
Image Analysis
General.
Activity and parametric PET images were coregistered to the participant’s T1-weighted anatomical MRI by using the FUSION tool in PMOD. MRI-based volumes of interest were determined on the T1-weighted MRI by using the maximum probability atlas and the brain parcellation tools of the NEURO tool in PMOD. The volumes of interest were transferred to the coregistered PET and T1rho images. Measures from these volumes of interest (as implemented by the NEURO tool in PMOD) were used for comparative analyses. Individual cortical volumes were calculated by summing the left and right hemispheric cortical volumes as determined by the brain parcellation tool.
FDG processing.
The FDG data were summed from 30 to 60 minutes and converted to standardized uptake value units (SUVs). FDG images were analyzed by volumes of interest based on participants’ structural MRI as well as by using a software tool designed specifically for the analysis of FDG images and assessing the risk of AD (Alzheimer’s discrimination analysis tool [PALZ]).
[11C]PiB imaging.
Amyloid burden was determined by using methods described by Price et al. (34) and Lopresti et al. (35). The distribution volume ratio (DVR) was calculated for each region on the basis of the fit, employing the simplified reference tissue method 2 as implemented by the kinetic tool (PMOD PKIN) using the nonspecific retention in the reference region (bilateral cerebellar gray matter) and the 90 minutes of data. In addition, the simple standardized uptake value ratios (SUVRs) were calculated for each region by using the SUV for the interval from 50 to 70 minutes postadministration and the bilateral cerebellar gray matter as the reference tissue.
The primary outcome variable from the [11C]PiB component of the study was the composite measure of amyloid burden (based on the volume-weighted mean of frontal, parietal, lateral temporal, anterior, and posterior cingulate regions), referred to as a cortical retention ratio, and regional measures of amyloid burden derived from the DVR and SUVR. These values each had corresponding global and regional measures of glucose metabolism (i.e., FDG SUV) and MRI-based T1rho values (global and regional mean and median T1rho values).
T1rho MRI processing.
The volumetric T1rho images were acquired by using a coronal three-dimensional fast low-angle shot sequence as described above. The T1rho relaxation times were calculated by fitting the voxel intensities versus the spin-lock time using a monoexponential signal decay model. The resulting relaxation time maps were coregistered with the T1-weighted anatomical images, and the volumes of interest were defined as described above. For each region, the mean and the median values were available. Because of the potential of CSF spillover unduly influencing the mean value, the median value for each region was also investigated. The global T1rho mean and median values were derived by calculating a volume-weighted average of the T1rho values for all intracerebral pixels as determined from the coregistered structural MRI. Because of the potential for incomplete coverage of the entire brain with the MRI field of view, regional comparisons and global calculations were limited to regions that had a minimum pixel value >0.
Statistical Analysis
The outcome variables of interest were the global mean T1rho and the global median T1rho scores. By using linear regression models, the relationships of age with both outcome variables were evaluated, followed by evaluations of the relationships between the imaging-based variables with T1rho, both mean and median, after adjustment for age. None of the variables had a significant interaction with age, and thus only main effects are reported. The R2 is reported for each model, as well as the increase in R2 over the age-only model. Then, to assess which regions of the brain showed relationships between T1rho measures (mean and median) and cognitive status (i.e., cognitively normal [HC] or cognitively abnormal [MCI or AD]), separate regression models for each region were run, controlling for age. To adjust for the evaluation of multiple models, the p values were adjusted by using the false discovery rate (36). Analyses were carried out with SAS/Stat software, version 9.4.
Results
Demographic Characteristics
Twenty-seven community-dwelling participants (men, N=13; women, N=14 [mean age=71.8 years [SD=11.3], range, 55–90 years]) underwent analyses. Cognitively, 17 participants were classified as HCs, seven as having MCI (six with early MCI and one with late-stage MCI), and three as having mild AD, yielding a total of 10 participants with cognitive impairment. Complete data were available for 11 of 12 participants with FDG imaging data. One participant with AD was unable to undergo the PiB imaging component because he moved away from the study location. Data for this participant were included in the analyses when available. Participants with AD and the one participant with late-stage MCI were all men. In the FDG cohort, the participant with late-stage MCI was the only subject with type II diabetes. Demographic characteristics and neuropsychological and global image-based measures are presented in Table 1.
Healthy control group (N=17) | Cognitively impaired group (N=10) | ||||||
---|---|---|---|---|---|---|---|
Parameter | Mean | SD | Range | Mean | SD | Range | pb |
Age (years) | 70.9 | 10.9 | 55–87 | 73.2 | 12.4 | 55–90 | 0.64 |
MMSE | 29.6 | 0.6 | 28–30 | 27.0 | 2.7 | 22–30 | 0.15 |
Trail-Making Test, Part B minus Part A (seconds) | 36.9 | 16.7 | 7–70 | 61.4 | 32.4 | 20–125 | 0.47 |
Logical memory–immediate (words) | 14.6 | 3.0 | 7–19 | 8.1 | 3.8 | 3–15 | 0.0003 |
Logical memory–delayed (words) | 14.1 | 2.6 | 8–19 | 5.0 | 2.9 | 0–8 | <0.0001 |
FDG-PET score (PALZ, from PMOD) | 0.43 | 0.23 | 0.28–0.76 | 1.12 | 0.93 | 0.24–3.03 | 0.08 |
Cortical retention ratio (distribution volume ratio) | 1.1 | 0.1 | 1.0–1.5 | 1.4 | 0.4 | 1.0–2.1 | 0.05 |
Cortical retention ratio (standardized uptake value ratios) | 1.2 | 0.2 | 1.1–1.8 | 1.5 | 0.4 | 1.1–2.5 | 0.05 |
Global mean T1rho (seconds) | 89.5 | 8.2 | 78.3–106.7 | 103.5 | 11.9 | 84.8–123.6 | 0.005 |
Global median T1rho (sec) | 81.8 | 5.1 | 75.3–94.8 | 90.7 | 9.7 | 79.0–111.7 | 0.02 |
Cortical volume (cc) | 456 | 71 | 340–583 | 411 | 62 | 332–496 | 0.10 |
TABLE 1. Demographic, neuropsychological, and global image-based parameters of cognitively normal participants (healthy control subjects) and participants with cognitive impairmenta
Relationships Between T1rho and Global Measures
The relationship between the global mean and global median T1rho values and neuropsychological and functional-pathological variables are presented in Table 2. Age was a highly significant predictor of the global mean and median T1rho values, accounting for >30% of the variance. Therefore, age was factored in the balance of the comparisons. With age in the model, the addition of other relevant AD-associated parameters improved the explanation of the variance in T1rho by 17%−42%. Higher T1rho values were associated with a clinical diagnosis of cognitive impairment (i.e., MCI or AD diagnosis), poorer neuropsychological test performance (i.e., lower Mini-Mental State Examination [MMSE] scores, poorer logical memory [both immediate and delayed], and longer times to complete the Trail-Making Test, Part B minus Part A), more abnormal glucose metabolism (i.e., higher scores on the PALZ), and higher amyloid burden (i.e., cortical retention ratio based on DVRs or SUVRs) (Table 2 and Figure 1).
Parametera | Equationb | Directionc | Parameter p | R2 | Change in R2 with parameter |
---|---|---|---|---|---|
Global mean T1rho (seconds) | |||||
Age (years) only | 49.3+0.63 age | + | 0.0008 | 0.37 | |
HC versus MCI_AD | 54.8+0.58 age–6.4 [HC] | – | 0.0002 | 0.65 | 0.28 |
MMSE | 139.1+0.60 age–3.0 MMSE | – | 0.0001 | 0.66 | 0.29 |
Logical memory–immediate (LMI) | 65.7+0.59 age–1.1 LMI | – | 0.006 | 0.54 | 0.17 |
Logical memory–delayed (LMD) | 61.3+0.65 age–1.3 LMD | – | <0.0001 | 0.68 | 0.31 |
TMT B–A | 42.3+0.58 age+0.24 TMTB–A | + | 0.0002 | 0.65 | 0.28 |
FDG PET score (PALZ) (N=12) | 41.6+0.68 age+10.3 PET | + | 0.001 | 0.82 | 0.42d |
CRR (DVR) | 22.5+0.63 age+23.0 CRR (DVR) | + | 0.0003 | 0.65 | 0.28 |
CRR (SUVR) | 25.8+0.62 age+18.6 CRR (SUVR) | + | 0.0005 | 0.65 | 0.28 |
Global median T1rho (seconds) | |||||
Age (years) only | 55.4+0.41 age | + | 0.002 | 0.32 | |
HC versus MCI_AD | 58.9+0.38 age–4.0 (HC) | – | 0.002 | 0.55 | 0.23 |
MMSE | 122.5+0.39 age–2.3 MMSE | – | <0.0001 | 0.65 | 0.33 |
Logical memory–immediate (LMI) | 65.9+0.38 age–0.69 LMI | – | 0.018 | 0.47 | 0.15 |
Logical memory–delayed (LMD) | 63.1+0.43 age–0.80 LMD | – | 0.0009 | 0.58 | 0.26 |
TMT B–A | 50.8+0.38 age+0.16 TMTB–A | + | 0.001 | 0.57 | 0.25 |
FDG-PET score (PALZ) (N=12) | 44.6+0.50 age+9.0 PET | + | 0.0002 | 0.87 | 0.52d |
CRR (DVR) | 34.6+0.39 age+19.1 CRR (DVR) | + | <0.0001 | 0.69 | 0.37 |
CRR (SUVR) | 37.4+0.39 age+15.4 CRR (SUVR) | + | <0.0001 | 0.68 | 0.36 |
TABLE 2. Relationship between T1rho measures and neuropsychological testing and imaging-based parameters, with control for age
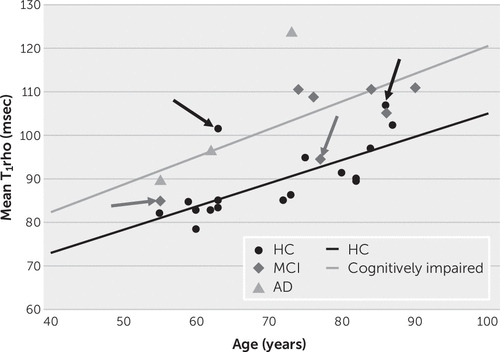
FIGURE 1. Relationships between global mean T1rho (msec) and age (years), by cognitive statusa
a Participants with impaired cognition (mild cognitive impairment [MCI] and Alzheimer’s disease [AD]) had a mean global T1rho of 56.8+0.64×age (R2=0.44, p=0.036). Healthy comparison subjects (HCs; normal cognition) had a mean global T1rho of 51.6+0.53×age (R2=0.51, p=0.0014). Arrows pointed toward circles indicate HCs with T1rho values more consistent with values for participants with cognitive impairments. Arrows pointed toward diamonds indicate participants with MCI with T1rho values more consistent with values for HCs.
Figure 2 shows these relationships graphically in the form of contour plots of the relationship between T1rho and selected pathological measures. Note the increased T1rho values with greater pathological burden and associated poorer cognition. Increasing age (far-right contours) did not exhibit the progressive decline in MMSE scores that was observed with increasing pathologies (cortical retention ratio [far left] or PALZ scores [middle contours]).
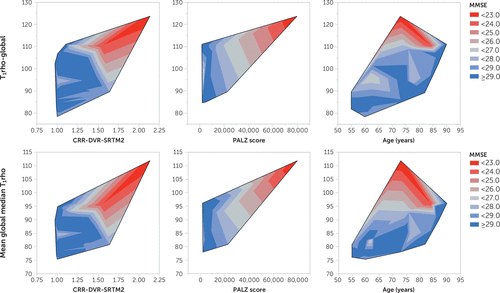
FIGURE 2. Contour plots of T1rhoa
a The graphs show global T1rho (global volume-weighted mean of regional mean values) (upper row) and mean global median T1rho (global volume-weighted mean of regional median values) (lower row) versus amyloid burden (expressed as the cortical retention ratio [CRR] calculated from the distribution volume ratio [DVR]) employing the simplified reference tissue method 2 (SRTM2) (left column), the ([18F]fluorodeoxyglucose (FDG)-based Alzheimer’s Discrimination Tool score (PALZ, from PMOD) (middle column), and age (years) contoured by the Mini-Mental State Examination (MMSE) score (red<gray<blue). Note the trend toward higher T1rho with lower MMSE (red) in all plots, with corresponding higher amyloid burden (higher CRR) and more aberrant glucose metabolism (higher PALZ score) but not necessarily greater age.
Cortical volume, as a surrogate for neurodegeneration, was significantly related to age (r=−0.67, p=0.0001) and to the T1rho measures (mean, r=−0.63, p=0.0005; median, r=−0.63, p=0.0004). With age and T1rho in the model, cortical volume was not significantly related to any of the neuropsychological or pathological measures, with p values ranging from 0.13 to 0.77. Therefore, T1rho was a stronger predictor than cortical volume for AD-associated neuropsychiatric measures.
Relationships Between T1rho and Regional Measures
The relationship between regional T1rho measures (mean and median) and cognitive status, controlled for age, is presented in Table 3. Of the 78 available regions for analysis, the regions reported are limited to those for which both age and the status variable were statistically significant. Regions for which the cognitive status p value remained significant after the false discovery rate correction are presented in bold in Table 3. In all cases, HCs exhibited a lower T1rho value than participants with cognitive impairment. It is noteworthy that except for the bilateral thalami and the right insula (which were no longer statistically significant after the false discovery rate correction), all significant regions were located in the frontal, cingulate, temporal, or parietal lobes, areas known to have significant amyloid deposition and to be selectively metabolically compromised in AD.
Regional T1rho median | Regional T1rho mean | |||||||
---|---|---|---|---|---|---|---|---|
Regionb | Direction (HC)c | p | p (FDR) | R2 | Direction (HC)c | p | p (FDR) | R2 |
Frontal gyrus, inferior–left | – | 0.007 | 0.039 | 0.49 | – | 0.016 | 0.10 | 0.58 |
Frontal gyrus, inferior–right | – | 0.002 | 0.031 | 0.59 | – | 0.0009 | 0.041 | 0.70 |
Frontal gyrus, middle–left | – | 0.006 | 0.039 | 0.52 | ||||
Frontal gyrus, middle–right | – | <0.0001 | <0.0001 | 0.74 | – | 0.012 | 0.094 | 0.45 |
Frontal precentral gyrus–left | – | 0.021 | 0.056 | 0.53 | ||||
Frontal precentral gyrus–right | – | 0.012 | 0.051 | 0.62 | – | 0.019 | 0.11 | 0.66 |
Frontal gyrus, superior–right | – | 0.047 | 0.099 | 0.65 | ||||
Cingulate gyrus, posterior–left | – | 0.0006 | 0.014 | 0.53 | – | 0.0022 | 0.051 | 0.53 |
Cingulate gyrus, posterior–right | – | 0.0068 | 0.039 | 0.39 | – | 0.022 | 0.11 | 0.41 |
Temporal gyrus, superior posterior–right | – | 0.02 | 0.031 | 0.47 | ||||
Temporal lobe, posterior–right | – | 0.0048 | 0.074 | 0.87 | ||||
Insula, right | – | 0.013 | 0.051 | 0.53 | – | 0.048 | 0.14 | 0.58 |
Parietal lobe, postcentral gyrus–left | – | 0.010 | 0.048 | 0.63 | ||||
Parietal lobe, postcentral gyrus–right | – | 0.017 | 0.053 | 0.61 | ||||
Parietal lobe, inferiolateral–left | – | 0.004 | 0.039 | 0.55 | – | 0.036 | 0.12 | 0.57 |
Parietal lobe, inferiolateral–right | – | 0.0046 | 0.039 | 0.55 | – | 0.0066 | 0.076 | 0.62 |
Parietal gyrus, superior–left | – | 0.010 | 0.048 | 0.48 | ||||
Parietal gyrus, superior–right | – | 0.045 | 0.099 | 0.49 | ||||
Thalamus–left | – | 0.017 | 0.053 | 0.37 | – | 0.031 | 0.11 | 0.36 |
Thalamus–right | – | 0.046 | 0.099 | 0.34 | – | 0.028 | 0.11 | 0.35 |
Corpus callosum | – | 0.038 | 0.12 | 0.34 | ||||
Caudate nucleus–left | – | 0.05 | 0.10 | 0.33 | – | 0.024 | 0.11 | 0.44 |
Caudate nucleus–right | – | 0.012 | 0.094 | 0.40 |
TABLE 3. Relationship between regional T1rho measures (median and mean) and cognitive status, with control for agea
Discussion
Currently, there are no disease-modifying therapies available for AD. Increasing evidence points toward the necessity of treating AD in the presymptomatic stage rather than waiting for the advent of a specific threshold initiation of a pathological cascade. However, the ability to diagnose AD depends on diagnostic tests performed in isolation, and for a given level of cognitive impairment, the biomarker profile can be markedly different between individuals (37). An in vivo measure that characterizes the brain microenvironment or pathological burden, such as T1rho, has the potential to provide a means to place an individual on the pathological continuum and to follow the trajectory over time better than single-function or pathology-oriented measures. Furthermore, this measure can be used serially without concern for the accumulated radiation burden associated with FDG, amyloid, and tau imaging.
It was found that T1rho increased with age and with increasing AD-associated pathologies. This observation is consistent with the reported relationship between age on brain pH. Bonnet et al. (29) reported a correlation between age and pH in the middle temporal gyrus of r=−0.68. In the present study, the correlation between age and T1rho (inversely related to pH) was r=0.57 and r=0.53 in the left and right superior posterior temporal gyrus, respectively, and 0.88 and 0.82 in the left and right posterior temporal lobe, respectively. Therefore, the relationship between age and a surrogate for pH (i.e., T1rho) was comparable to the direct relationship observed between age and pH. Beyond this relationship and consistent with the role of acidosis in the pathological cascades, after the analysis controlled for age, T1rho increased with impaired cognition (decreasing MMSE scores and poorer immediate and long-term memory scores), increasing amyloid burden (based on PiB-based cortical retention ratios), and more aberrant glucose metabolism (based on FDG-based metabolism).
Previous work exploring the potential utility of T1rho imaging in AD has exhibited promising results but has been hampered by limitations regarding the extent of brain characterized (e.g., medial temporal lobe only) and the classification of study subjects and pathologies (e.g., clinical diagnoses only). Borthakur et al. (20) examined the T1rho signal in medial temporal lobe gray and white matter in participants with AD, compared with those with MCI and with HCs. On average, participants with AD had a 6% increase in T1rho, compared with HCs (8% for white matter and 5% for gray matter), with participants with MCI having an intermediate mean value. The HC and MCI groups tended to show a somewhat bimodal distribution in white matter T1rho values, assumed to be due to existing premorbid pathology in some study subjects in these groups. Participant classification was based on neuropsychological tests, not knowledge of AD-specific pathologies. Although positive change in T1rho in white matter and gray matter was exhibited with age, neither relationship was statistically significant. In our study, in which age was found to be a statistically significant factor, a 70-year-old individual with cognitive impairment would exhibit global mean and median T1rho values that were 7.2% and 4.9% higher, respectively, compared with their HC counterparts, values consistent with the differences reported in the literature.
Gray matter and white matter T1rho in the medial temporal lobe in participants with AD or with MCI and in an age-matched control group were examined by Haris et al. (22). T1rho values in gray matter and white matter, respectively, were lowest in the HC group (87.5 ms [SD=1.2] and 80.5 ms [SD=1.4]), intermediate in the MCI group (90.9 ms [SD=1.3] and 84.1 ms [SD=2.7]), and highest in the AD group (91.9 ms [SD=0.8] and 88.3 ms [SD=1.3]). These differences were statistically significant between participants with AD and HCs but not between participants in the MCI and AD groups or between participants in the MCI and HC groups. No significant relationships between age and T1rho and between MMSE scores and T1rho were observed. Five of the HCs had significantly increased T1rho in both gray and white matter.
Haris et al. (21) also examined the hippocampal (right and left) T1rho in control subjects and in patients with AD, PD, or PDD. T1rho was significantly increased in patients with AD compared with both HCs and patients with PD but was not significantly different from patients with PDD. T1rho was decreased in patients with PD compared with control subjects and patients with PDD. In contrast to the present study, no significant correlations were observed between T1rho and age or T1rho and MMSE scores when the analysis was limited to the hippocampal areas only. However, the authors concluded that serial T1rho imaging may provide insight into AD and PD disease progression and may assist in the early diagnosis of these diseases.
In addition, Haris et al. (23) evaluated the sensitivity and specificity of the combination of T1rho of the white matter and gray matter in the medial temporal lobe in combination with CSF biomarkers (β-amyloid1–42; T-tau and P-tau, 181 p levels) for categorizing individuals with AD or with MCI and in control subjects who were classified on the basis of a clinical consensus diagnoses. Using binary logistic regression, the combination of T1rho and CSF biomarkers predicted 86.4% of control subjects and 66.7% of participants with MCI for the comparison between the control and MCI groups and 59.3% of MCI participants and 84.6% of AD participants for the comparison between the MCI and AD groups. Receiver-operating characteristic analyses revealed that T1rho had greater sensitivity (HC group versus MCI group, 0.60 versus 0.53; HC group versus AD group, 0.82 versus 0.77, for T1rho and CSF biomarkers, respectively) and CSF biomarkers had greater specificity (HC group versus MCI group, 0.77 versus 0.82; HC group versus AD group, 0.71 versus 0.79, for T1rho and CSF biomarkers, respectively) in distinguishing participants with MCI or AD from HCs. The investigators did not find any significant correlations between T1rho and CSF biomarkers or between T1rho and age. They concluded that the combination of T1rho and CSF biomarkers showed promise as a specific and early diagnostic measure for AD and may easily track the progression from MCI to AD.
In line with the literature, significant differences were found between the clinical diagnostic classifications and T1rho values (HCs lower than participants with cognitive impairment); however, by expanding the T1rho field of view beyond the hippocampus and medial temporal lobe, the relationships between global T1rho and age and global T1rho and MMSE scores were discernible. Follow-up is needed to discern whether these differences translate to altered risk of deterioration. Analyses of regional T1rho values revealed significant relationships only marginally with the medial temporal structures but primarily with frontal, parietal, and cingulate structures, areas known to have AD-associated pathology (glucose hypometabolism and amyloid deposition).
T1rho MRI is a pH-sensitive measure, with higher values consistent with a more acidic environment. The overall consistency of higher T1rho values with greater cognitive impairment and pathological burden is concordant with the development of acidosis as an integral component of the various AD-associated pathological cascades as described by Humpel (1). Having a marker that varies according to a feature common to each of the cascades rather than one unique only to a particular pathology presents the possibility of placing an individual on a pathological trajectory rather than exhibiting a constellation of biomarker values for a particular level of impairment (37). T1rho MRI holds promise as a potentially useful biomarker providing unique information beyond the T1-weighted measures of neurodegeneration.
However, our study has several limitations that warrant further investigation before the full utility of T1rho MRI can be determined. First, it should be noted that T1rho imaging is not exclusively sensitive to pH, and other factors, such as glucose (38) and glutamate (5) concentrations, have been shown to influence T1rho relaxation times at higher magnetic fields and spin-lock amplitudes. In AD, there are known changes in protein aggregates and in glutamate and glucose concentrations that could influence T1rho relaxation times. Thus, it cannot be ruled out that other metabolites may be driving the T1rho relaxation time changes observed in this study. However, recent phantom work conducted by Owusu et al. (11) revealed that the T1rho signal was more sensitive to changes in physiologic pH than to changes in glucose or lysine concentrations in a protein-rich medium (egg-white albumin phantom) at physiologic temperatures, adding assurance that the observed in vivo T1rho signal likely reflects pH. Second, it is currently unknown whether there are dispersion effects of the spin-lock pulse in vivo that would vary its sensitivity across regions of the brain. Future studies might utilize several spin-lock amplitudes to evaluate dispersion effects and regional sensitivity. Third, our study sample was small and skewed toward HCs, with participants with AD limited to the lower half of the age range only. A broader range of older participants with more extensive pathology will be needed to fully explore the interactions between age, cognitive impairment, and pathological burden.
Fourth, and most important, this study was cross-sectional only. In order for T1rho MRI to be an effective metric of AD-associated pathology, it needs to be reproducible (i.e., produce the same values on day 1 compared with day 2), reliable (i.e., exhibit the same relationship between the T1rho measure, cognition, and other pathological measures at time 1 and time 2), and longitudinally consistent (i.e., on an individual basis, increasing T1rho would equal decreasing cognitive performance; stable T1rho would equal stable cognition). Reproducibility data were not available for our study population and scanner configuration. However, recent investigations conducted at this institution (data available upon request from Dr. Laura L. Boles Ponto) using a state-of-the-art, high-resolution, efficient sagittal segmented three-dimensional gradient echo sequence (TE=2.5 ms, TR=5.6 ms, field of view=220×220×160 mm3, matrix=128×128×80, flip angle=10°, and acceleration=2, spin-lock amplitude=400 Hz, spin-lock durations=0 and 80 ms) implemented on a 3.0-T GE Discovery 750W MRI scanner revealed that for a limited group of study subjects (N=5), T1rho values were highly reproducible in the short-term (i.e., 7–14 days) both regionally and globally. For a combination of subcortical and cortical hemispheric regions (N=20 per subject), the correlations between time 1 and time 2 were 0.90 and 0.92, respectively, with average differences of –1.7% and –1.1% for mean and median values, respectively. Data are currently not available to address the reliability and longitudinal consistency of these measures but are the basis of future T1rho-related research at this institution.
Conclusions
T1rho MRI, a pH-sensitive measure, was significantly associated with age and clinical status (HCs compared with participants with cognitive impairment). After controlling for age, global T1rho MRI was positively associated with poorer cognition (lower MMSE score) and memory (lower immediate and delayed memory scores), greater amyloid burden (based on [11C]PiB SUVRs and DVRs), and more abnormal glucose metabolism. Regional T1rho MRI values in frontal and cingulate regions, after controlling for age, were positively associated with cognitive impairment. T1rho shows promise as a metric of AD-associated pathology, potentially providing a single measure for assessing disease status and for monitoring the trajectory of the disease over time or with therapy.
1 : Chronic mild cerebrovascular dysfunction as a cause for Alzheimer’s disease? Exp Gerontol 2011; 46:225–232Crossref, Medline, Google Scholar
2 : Mitochondria and cell bioenergetics: increasingly recognized components and a possible etiologic cause of Alzheimer’s disease. Antioxid Redox Signal 2012; 16:1434–1455Crossref, Medline, Google Scholar
3 : Oxidative stress and amyloid-beta pathology in normal individuals with a maternal history of Alzheimer’s. Biol Psychiatry 2010; 68:913–921Crossref, Medline, Google Scholar
4 : Role of hyperglycaemia-related acidosis in ischaemic brain damage. Acta Physiol Scand 1997; 161:567–580Crossref, Medline, Google Scholar
5 : Characterization of non-hemodynamic functional signal measured by spin-lock fMRI. Neuroimage 2013; 78:385–395Crossref, Medline, Google Scholar
6 : Effects of intracellular pH, blood, and tissue oxygen tension on T1ρ relaxation in rat brain. Magn Reson Med 2002; 48:470–477Crossref, Medline, Google Scholar
7 : Detecting activity-evoked pH changes in human brain. Proc Natl Acad Sci USA 2012; 109:8270–8273Crossref, Medline, Google Scholar
8 : Estimation of the onset time of cerebral ischemia using T1ρ and T2 MRI in rats. Stroke 2010; 41:2335–2340Crossref, Medline, Google Scholar
9 : Proton exchange as a relaxation mechanism for T1 in the rotating frame in native and immobilized protein solutions. Biochem Biophys Res Commun 2001; 289:813–818Crossref, Medline, Google Scholar
10 : Low spin-lock field T1 relaxation in the rotating frame as a sensitive MR imaging marker for gene therapy treatment response in rat glioma. Radiology 2007; 243:796–803Crossref, Medline, Google Scholar
11 : R1ρ sensitivity to pH and other compounds at clinically accessible spin-lock fields in the presence of proteins. NMR Biomed 2020; 33:
12 : Evaluation of activity-dependent functional pH and T1ρ response in the visual cortex. Neuroimage 2014; 95:336–343Crossref, Medline, Google Scholar
13 : Eccentricity mapping of the human visual cortex to evaluate temporal dynamics of functional T1ρ mapping. J Cereb Blood Flow Metab 2015; 35:1213–1219Crossref, Medline, Google Scholar
14 : Rapid acquisition strategy for functional T1ρ mapping of the brain. Magn Reson Imaging 2014; 32:1067–1077Crossref, Medline, Google Scholar
15 : Functional t1ρ imaging in panic disorder. Biol Psychiatry 2014; 75:884–891Crossref, Medline, Google Scholar
16 : Brain abnormalities in bipolar disorder detected by quantitative T1ρ mapping. Mol Psychiatry 2015; 20:201–206Crossref, Medline, Google Scholar
17 : Alterations of the cerebellum and basal ganglia in bipolar disorder mood states detected by quantitative T1ρ mapping. Bipolar Disord 2018; 20:381–390Crossref, Medline, Google Scholar
18 : T1ρ imaging in premanifest Huntington disease reveals changes associated with disease progression. Mov Disord 2015; 30:1107–1114Crossref, Medline, Google Scholar
19 : Early marker for Alzheimer’s disease: hippocampus T1rho (T(1rho)) estimation. J Magn Reson Imaging 2009; 29:1008–1012Crossref, Medline, Google Scholar
20 : T1ρ MRI of Alzheimer’s disease. Neuroimage 2008; 41:1199–1205Crossref, Medline, Google Scholar
21 : T1rho (T1ρ) MR imaging in Alzheimer’s disease and Parkinson’s disease with and without dementia. J Neurol 2011; 258:380–385Crossref, Medline, Google Scholar
22 : T(1ρ) MRI in Alzheimer’s disease: detection of pathological changes in medial temporal lobe. J Neuroimaging 2011; 21:e86–e90Crossref, Medline, Google Scholar
23 : T1rho MRI and CSF biomarkers in diagnosis of Alzheimer’s disease. Neuroimage Clin 2015; 7:598–604Crossref, Medline, Google Scholar
24 : Multi-modal brain MRI in subjects with PD and iRBD. Front Neurosci 2017; 11:709Crossref, Medline, Google Scholar
25 : T1ρ and T2ρ MRI in the evaluation of Parkinson’s disease. J Neurol 2010; 257:964–968Crossref, Medline, Google Scholar
26 : T1ρ relaxation time in brain regions increases with ageing: an experimental MRI observation in rats. Br J Radiol 2016; 89:
27 : Age or ischemia uncouples the blood flow response, tissue acidosis, and direct current potential signature of spreading depolarization in the rat brain. Am J Physiol Heart Circ Physiol 2017; 313:H328–H337Crossref, Medline, Google Scholar
28 : In vivo whole-brain T1-rho mapping across adulthood: normative values and age dependence. J Magn Reson Imaging 2014; 40:376–382Crossref, Medline, Google Scholar
29 : Aging is associated with a mild acidification in neocortical human neurons in vitro. J Neural Transm (Vienna) 2018; 125:1495–1501Crossref, Medline, Google Scholar
30 : Hypothesis on the relationship between the change in intracellular pH and incidence of sporadic Alzheimer’s disease or vascular dementia. Int J Neurosci 2010; 120:591–595Crossref, Medline, Google Scholar
31 : Alzheimer’s Disease Neuroimaging Initiative (ADNI): clinical characterization. Neurology 2010; 74:201–209Crossref, Medline, Google Scholar
32 . Synthesis and evaluation of 11C-labeled 6-substituted 2-arylbenzothiazoles as amyloid Imaging agents. J Med Chem 2003; 46:2740–2754Crossref, Medline, Google Scholar
33 : Artifacts in T(1rho)-weighted imaging: correction with a self-compensating spin-locking pulse. J Magn Reson 2003; 162:113–121Crossref, Medline, Google Scholar
34 : Kinetic modeling of amyloid binding in humans using PET imaging and Pittsburgh Compound-B. J Cereb Blood Flow Metab 2005; 25:1528–1547Crossref, Medline, Google Scholar
35 : Simplified quantification of Pittsburgh Compound B amyloid imaging PET studies: a comparative analysis. J Nucl Med 2005; 46:1959–1972Medline, Google Scholar
36 : Controlling the false discovery rate: a practical and powerful approach to multiple testing. J R Stat Soc B 1995; 57:289–300Google Scholar
37 : Tracking pathophysiological processes in Alzheimer’s disease: an updated hypothetical model of dynamic biomarkers. Lancet Neurol 2013; 12:207–216Crossref, Medline, Google Scholar
38 : Mapping brain glucose uptake with chemical exchange-sensitive spin-lock magnetic resonance imaging. J Cereb Blood Flow Metab 2014; 34:1402–1410Crossref, Medline, Google Scholar