Sensitization Phenomena in Psychiatric Illness
Abstract
Sensitization or “kindling-like” phenomena have been implicated in the pathophysiology of a number of psychiatric illnesses. A basic understanding of the prototypical sensitization phenomenon, the kindling model of epilepsy, is thus of increasing significance for the psychiatrist. This article presents a summary of the kindling model, with particular emphasis on glutamatergic mechanisms in general and plasticity of the N-methyl-d-aspartate (NMDA) receptor in specific. Findings from the kindling model are then discussed in light of their potential relevance to psychiatric illness. Finally, a speculative model is proposed in which opposing molecular processes lead to NMDA receptor hyperfunction in kindling and hypofunction in schizophrenia.
Sensitization or “kindling-like” phenomena have received a great deal of attention in efforts to conceptualize the pathophysiology and natural history of psychiatric disorders. Pathophysiological models of psychiatric diseases as seemingly diverse as mood disorders,1–5 schizophrenia,6–9 drug addiction,10–14 posttraumatic stress disorder,15,16 multiple chemical sensitivity,17,18 and pain19–21 have all implicated sensitization mechanisms. The term sensitization refers to the development over time of an exaggerated, pathological response to a stimulus that was originally innocuous or “subthreshold” (i.e., the stimulus initially did not elicit an unusual behavior or physiological response). In order for sensitization to develop, a given stimulus must be repeated over time. Once the pathological response has developed to the stimulus, it is thought to persist for the lifetime of the organism. The term kindling originally referred to a very specific type of sensitization process: the development of behavioral seizures in response to repeated electrical or chemical stimuli. The word kindling is now more widely used to convey the concept of the development of persistent hypersensitivity to a given stimulus.
The importance of sensitization phenomena in current psychiatric thought makes a basic understanding of kindling imperative. Likewise, an understanding of some of the molecular substrates identified in the kindling model of epilepsy should serve to assist in the development of hypotheses regarding sensitization mechanisms in psychiatric illness. Thus, the purpose of this article is to review the kindling paradigm as it relates to the development of behavioral seizures in rodents, and, more specifically, to examine the role of N-methyl-d-aspartate (NMDA) receptors in the development and expression of kindling-induced hyperexcitability. The article begins with a brief description of the kindling model and a rationale for the study of the hippocampus in this model, followed by background on glutamate receptors in general and NMDA receptors in specific. The role of NMDA receptors in the development and expression of kindled seizures is discussed next, and then studies of NMDA receptor plasticity in the kindling model are reviewed. Finally, principles derived from these studies are extended to sensitization phenomena in general, with particular emphasis on psychiatric illness.
THE KINDLING MODEL OF EPILEPSY
Kindling is the most widely studied animal model of complex partial epilepsy. In this model, an initially subconvulsive stimulus is periodically administered to the brain of an animal (most often a rodent); over time, this same stimulus results in intense limbic and clonic motor seizures.22–24 Sevillano first described this effect in 1961, discovering that repeated administration of low levels of electrical current to the hippocampus resulted in progressive intensification of stimulus-induced seizure activity.25 Goddard subsequently recognized the importance of this phenomenon, and he named it as well, using the term kindling by analogy to the lighting of a fire.25,26 The stimulus is usually a small current (100 to 1,000 μA) delivered through an electrode that has been stereotactically implanted into a specific brain region, most often the amygdala. The initial stimulus elicits little change in behavior or electrical brain activity. Additional stimulations result in focal after-discharge (AD) or electrical seizure recorded with an electroencephalogram (EEG). Initially, the AD is not accompanied by overt behavioral seizures. However, subsequent stimulations induce progressive lengthening and propagation of the AD into brain nuclei that underlie behavioral seizures. The intensity of the behavioral seizures increases over the course of kindling in a stereotypical manner, (i.e., facial clonus → head nodding → contralateral forelimb clonus → rearing → rearing and falling)27 with animals ultimately displaying tonic-clonic motor seizures.
THE HIPPOCAMPUS: A MODEL FOR THE STUDY OF THE MOLECULAR MECHANISMS OF KINDLING
The hippocampus has been a focus in kindling research for several reasons. First, it is this structure, along with other parts of the limbic system, that has been most strongly implicated as the focus of abnormal neuronal activity in the majority of human complex partial seizures.28,29 Indeed, pathological changes in hippocampal structures (such as mesial temporal sclerosis, in which neuronal loss occurs in hippocampal subfields, particularly fascia dentata and CA1) are often identified in tissue excised for the treatment of drug-resistant complex partial epilepsy.29 Second, the circuitry of the principal neurons of the hippocampus is well established, and thus potential alterations in biochemical or physiological properties of subtypes of hippocampal neurons can be appreciated in light of their impact on information flow through this circuit (Figure 1). And third, the hippocampal slice preparation has a distinct advantage in that some of the major synaptic pathways connecting the principal neuronal populations remain intact following dissection and sectioning (Figure 1). Thus, electrophysiological properties of both control and experimental neurons can be studied in a microenvironment that approximates at least some of the network connections that occur in vivo.
Measurements of neurotransmitter receptor density and neuroanatomical lesion studies have implicated the hippocampus as an important structure in kindling. Early studies of the hippocampus using radioligand binding assays provided molecular evidence for a modification, following kindling, of neurotransmitter receptors intrinsic to discrete populations of neurons.30 These studies identified changes in the density of muscarinic cholinergic receptors31 and benzodiazepine receptors32 on dentate granule cells.30,33,34 The importance of the hippocampus was further indicated by the observation that selective elimination of hippocampal dentate granule cells resulted in a marked slowing of the rate of kindling development.35,36 Likewise, disruption of the excitatory input into the hippocampus profoundly slowed the rate of kindling development.37 Results of these lesion analyses led to the conclusion that the hippocampus facilitates the formation of kindling in situ.
Electrophysiological studies of hippocampal slices in vitro showed that the hippocampus, in addition to facilitating the development of kindling, also displayed abnormal excitability after kindling developed. In slices examined one day or one month following the last kindled seizure, hyperexcitability was identified in all three principal neuronal populations of the hippocampus: the dentate granule cells, the CA2/3 pyramidal cells, and the CA1 pyramidal cells.38 These studies clearly implicated the hippocampus as one structure of the kindled brain having abnormal excitability. They also demonstrated the feasibility of detecting differences between control and kindled animals ex vivo, a necessary step for the analysis of the mechanisms of hyperexcitability.
GLUTAMATE RECEPTORS: A BRIEF PERSPECTIVE
Although it is generally accepted that synapses using glutamate and related excitatory amino acids represent the principal excitatory synapses in the mammalian central nervous system, it took many years of experimentation before this idea gained widespread approval. It was not until the studies of Curtis, Watkins, and colleagues that a putative role for this amino acid as a neurotransmitter was proposed. Iontophoretic application of glutamate concurrent with intracellular recording (by means of a double-barreled electrode) revealed membrane depolarization, an observation that suggested an interaction of these compounds with a membrane-associated receptor.39,40 Importantly, other analogues of glutamate, including N-methyl-dl-aspartate, could elicit membrane depolarization, and compounds that showed depressant effects, such as γ-aminobutryic acid (GABA), could antagonize depolarization.40 The identification of potent uptake systems that allowed glutamate to be cleared from the extracellular space helped explain how such a ubiquitous compound could serve a very selective role. However, the lasting acceptance of glutamate's role as a neurotransmitter did not result until the identification of subtypes of glutamate receptors.
Glutamate Receptor Subtypes: The NMDA Receptor
One of the most important developments implicating glutamate as a neurotransmitter came from evidence in the 1970s that different receptor types could be activated by this amino acid. Early evidence for glutamate receptor subtypes stemmed from differences in physiological responses produced by different agonists.41–43 An early classification defined NMDA and non-NMDA receptors, a concept that was solidified by the introduction of potent and selective antagonists, particularly for the NMDA receptor.44–49 Further pharmacological and electrophysiological studies revealed the existence of at least two types of non-NMDA receptors: the KA (kainate) and AMPA (α-amino-3-hydroxyl-5-methyl-4-isoxazole propionate) receptors. Subsequent radioligand binding studies supported a classification of glutamate receptors into at least three principal receptor subtypes: the NMDA, KA, and AMPA subtypes.49 These three glutamate receptor subtypes are termed ionotropic receptors because they allow ion flux upon ligand binding (as contrasted to the metabotropic or G-protein coupled glutamate receptors).
Of all the glutamate receptor subtypes the NMDA receptor is best characterized, because of the relatively large number of selective agonists and antagonists that recognize it. The receptor has multiple regulatory and pharmacological domains (Figure 2). The transmitter recognition site is bound by both agonists (e.g., glutamate, aspartate, NMDA) and competitive antagonists (e.g., APV [2-amino-5-phosphonovalerate], CPP [3-(2-carboxypiperazin-4-yl)-propyl-1-phosphonic acid], CGS 19755 [1-(cis-2-carboxypiperidine-4-yl)-propyl-1-phosphonic acid]). Binding of the co-agonist glycine to its site appears crucial for receptor activation.50–52 Dissociative anesthetics, such as PCP (phencyclidine), ketamine, MK-801 (dizocilpine), and TCP (1-(1-(2-thienyl)-cyclohexyl)-piperidine), act as uncompetitive antagonists by binding within the channel pore, at or near the Mg2+ binding site.53,54 Polyamines such as spermine have been shown to interact with and modulate channel function.55–58 Zinc appears to interact with the receptor and antagonize NMDA responses,59,60 possibly through inhibition of glycine binding.61 This list of NMDA receptor modulatory sites is by no means exhaustive; indeed, other sites have been described, including a redox regulatory site62,63 and a mechanosensitive site.64 The large number of regulatory sites suggests that NMDA receptor–mediated effects can be modulated in many subtle ways by several distinct endogenous and exogenous compounds.
In the brain, NMDA receptor–mediated responses are slow in onset and are of relatively long duration (as compared to the fast depolarizing responses mediated by AMPA receptors, the “workhorses” of glutamatergic neurotransmission). The NMDA receptor is permeable to calcium as well as to sodium and potassium.65–67 In addition to gating by glutamate and glycine, NMDA receptor activation is voltage-dependent secondary to a block of the channel pore by Mg2+ at more negative membrane potentials.68,69 Because of this voltage-dependent block, NMDA receptor activation occurs only when the neuron is partially depolarized, perhaps following activation of AMPA receptors.43,70 The influx of calcium through this receptor subtype and the fact that its activation is coupled to depolarization (i.e., activity-dependent) have implicated the NMDA receptor in neuronal plasticity, both in normal development and in neuropathology.
Subunits of Glutamate Receptor Subtypes
Our understanding of the molecular structure of glutamate receptors began 10 years ago, with the cloning by functional expression of a glutamate receptor by Hollmann et al.71 This feat initiated the cloning of representative members of the various subtypes of glutamate receptors. Members of AMPA,71–74 kainate,75–80 and NMDA81–87 receptor families have all been cloned. These families of receptors include multiple subunit types that may form homomeric (composed of only one subunit type) or heteromeric (composed of two or more different subunit types) receptors.88,89 Adding to the complexity is the fact that splice variants of subunit messenger ribonucleic acids (mRNAs) have been described for AMPA90 and NMDA91,92 receptor types. RNA editing has been described for some members of the AMPA and KA families.93,94 It is unclear at present which subunits within a family form functional receptor channel complexes in the intact animal, although recent evidence suggests that at least four subunits come together to form a functional receptor.95
Of particular interest are the NMDA receptor channel subunits. To date, five subunits have been described for both rat and mouse.83–87 The first subunit to be cloned, NMDAR1,81 is functional as a homomeric receptor channel. NMDAR1 displays a pharmacological profile similar to native receptors for NMDA receptor agonists and antagonists, as well as modulation by glycine and voltage-dependent Mg2+ block. The receptor mRNA is widely expressed in rat brain, with particularly marked expression in hippocampus. Four additional subunits comprising a related family of NMDA receptors have also been described.83–87 These subunits are known as NR2A–D in rat and ε1–4 in mouse. These subunits are nonfunctional when expressed alone (homomeric) or together (heteromeric); however, when expressed with NMDAR1, they alter the properties of the receptor channel complex. All show differential but sometimes overlapping patterns of expression. The genes expressed in adult rat hippocampus are NR2A and NR2B, with NR2C and NR2D showing little or no expression in the granule and pyramidal neurons of this area.96 Properties of the receptor channel complex that are subunit composition–dependent include magnitude of current flux with agonist stimulation, kinetics of current response to agonist, level of voltage-dependent Mg2+ block, and degree of inhibition by NMDA receptor antagonists. These findings, derived from recombinant cotransfection/coexpression experiments, suggest that native NMDA receptors may differ in their sensitivity to voltage-dependent Mg2+ block, agonists, and antagonists as a function of their subunit composition. Thus, functional diversity of native NMDA receptors may be expected from the assembly of different subunit combinations.
EVIDENCE IMPLICATING NMDA RECEPTORS IN SEIZURE ACTIVITY IN VITRO AND IN VIVO
Findings from in vitro studies suggest that the NMDA receptor participates in the generation of seizure activity, particularly in the expression of bursts of action potentials. As discussed above, activation of NMDA receptors is voltage-dependent because of the block of the channel by Mg2+ at more negative membrane potentials.66,68,69 At normal resting membrane potentials, Mg2+ blocks the channel and low concentrations of NMDA fail to evoke current. Following depolarization, the Mg2+ block of the channel is released and NMDA receptor agonists can effectively evoke current. The fact that the NMDA receptor is gated by both voltage and ligand endows NMDA receptor–mediated neurotransmission with associative and regenerative properties that can contribute to enhanced depolarization and burst firing similar to that observed in epileptiform discharges.68,97,98 Furthermore, in vitro studies of tissue slice preparations (examining the effects of NMDA receptor antagonism on seizure-like activity) have demonstrated that NMDA receptor–mediated neurotransmission can contribute to epileptiform activity.99
A correlate to the role of NMDA receptors in these in vitro models is the role of this receptor subtype in seizure expression in vivo. Several studies have shown that NMDA receptor antagonists are effective antiseizure drugs in models of acute seizures, including chemical and electroshock-evoked seizures.100–103 Additionally, NMDA receptor antagonists have been shown to be effective antiseizure drugs in genetic models of epilepsy.104–108
ENHANCED SYNAPTIC FUNCTION AS A MECHANISM OF THE HYPEREXCITABILITY IN KINDLING
Given the role of NMDA receptor–mediated responses in both in vitro and in vivo models of seizure activity, it is possible that enhanced function of synapses using this receptor is a mechanism underlying the enduring hyperexcitability of a “kindled” brain. If this is the case, then it could be expected that antagonists of the NMDA receptor subtype would inhibit kindled seizures. Many laboratories have tested this idea, and an overwhelming consensus has formed: NMDA receptor antagonists (both competitive and uncompetitive) do indeed inhibit kindled seizures; however, they are much less efficacious as antiseizure drugs than they are as antiepileptogenic drugs.103,109–112 That is, they are quite effective in inhibiting the development of kindling, but less effective in inhibiting seizures in fully kindled animals.
Thus, the lower antiseizure efficacy of NMDA receptor antagonists could suggest that NMDA receptors play a lesser role in the expression of the hyperexcitability in kindling. Alternatively, however, these findings could be explained by an enhancement of NMDA receptor expression and/or function during the kindling process; evidence for this idea is discussed below. Perhaps kindling alters NMDA receptors, making them more sensitive to endogenous excitatory amino acids (evidenced by hyperexcitability) while at the same time less sensitive to exogenous antagonists (evidenced by poor antiseizure efficacy of NMDA receptor antagonists).
Evidence From Dentate Granule Cells
Much experimental evidence exists to support the idea that NMDA receptor responses are augmented following kindling. Electrophysiological analyses of dentate granule cell response to synaptic stimulation in hippocampal slices from kindled animals first suggested that kindling induces a long-lasting enhancement of NMDA receptor–mediated activity. In these studies, stimulation of the lateral perforant path in hippocampal slices from kindled animals revealed an NMDA receptor component of the dentate granule cell excitatory postsynaptic potential (EPSP), a response not detected in control slices.113,114 Importantly, the expression of the NMDA receptor component was long lasting (at least 6 weeks after the last seizure), suggesting that it might contribute to the persistence of hyperexcitability of the kindled brain (but see also Sayin et al.115).
The expression of this NMDA receptor component of the EPSP may arise from alterations intrinsic to the NMDA receptor itself. Whole-cell patch clamp and single-channel recordings of acutely dissociated dentate granule cells have disclosed increases in mean channel open time as well as a reduced sensitivity to Mg2+ of NMDA receptors following kindling.116 They have also demonstrated that kindling results in an enhanced potency of NMDA at NMDA receptors on these dentate granule cells.117 The data indicate that kindling induces a population of NMDA receptors whose properties would be expected to enhance the response of dentate granule cells to synaptically released glutamate (i.e., to produce an increase in excitability). It appears likely that the NMDA receptor alterations identified by electrophysiological analysis of single channels on granule cells contribute to the expression of the NMDA receptor–mediated component of the granule cell EPSP following kindling.
Evidence From Pyramidal Cells
Biochemical and electrophysiological analyses of NMDA receptor function following kindling have suggested long-lasting alterations in this glutamate receptor subtype in hippocampal pyramidal cell populations as well. Morrisett et al.118 found that NMDA more potently depressed muscarinic cholinergic receptor–mediated phosphatidyl inositol (PI) turnover in hippocampal slices from kindled rats relative to control. This finding suggested that following kindling, NMDA more potently depolarized hippocampal neurons. However, this indirect measure of depolarization could not discern whether the enhanced NMDA potency was present throughout the hippocampus or was localized to a specific neuronal population. To localize the enhanced potency of NMDA to specific populations of hippocampal pyramidal cell neurons, NMDA-evoked depolarizations were studied in CA3 and CA1 of hippocampal slices from control and kindled animals by use of the grease-gap preparation.119 When studied either 24 hours or 1 to 2 months after the last kindled seizure, CA3 (but not CA1) pyramidal cells from kindled rats were five- to six-fold more sensitive to NMDA than those from control.120 Thus, studies using the grease-gap preparation indicate that the enhanced sensitivity to NMDA originally described in whole hippocampal slices was due to enhanced NMDA responsiveness of CA3 pyramidal cells.
Induction of Novel NMDA Receptors
One explanation for the increased sensitivity of CA3 pyramidal cells to NMDA is an increase in the receptor reserve in this population of neurons. Radioligand binding studies using whole hippocampal membranes indicated an increase in NMDA receptor number following kindling.121 Specifically, when binding was studied 28 days after the last kindled seizure, increases in the number of binding sites for a competitive NMDA receptor antagonist (CPP), for glycine, and for glutamate were detected.96,121 Surprisingly, no alterations in the binding of a second competitive antagonist, CGS 19755 (structurally quite similar to CPP), were detected in whole hippocampal membranes at this time point.122 Subsequently, antagonist-binding assays using membranes prepared from fascia dentata, CA3, and CA1 revealed that the increase in CPP binding was limited exclusively to CA3.96 The differential-binding properties of two distinct, though structurally similar, NMDA receptor antagonists indicated that kindling induced a novel population of NMDA receptors in hippocampal region CA3.96 Specifically, an increase in the number of CPP binding sites, but not CGS 19755 binding sites, was detected following kindling. Additionally, the affinity of CPP for the NMDA receptor was much lower for receptors isolated from CA3 hippocampal membranes of kindled animals than from control animals.96 This reduced affinity of CPP, as measured biochemically, predicted the reduced potency of CPP and similar competitive antagonists in inhibiting NMDA receptor responses when measured electrophysiologically.123
The Hypothesis of Altered Subunit Composition
Taken together, the studies described above indicate that kindling induces the expression of populations of novel NMDA receptors (termed NMDARK) in dentate granule cells and CA3, but not CA1, pyramidal cells. These receptors display novel functional and pharmacological properties. These kindling-induced changes likely reflect, at least in part, alterations intrinsic to the receptor itself. It is of interest that the magnitude of these changes (at least as measured using radioligand binding) increases over time. The predicted functional effects of NMDA receptor modulation in kindling are consistent with an enhanced response to neurotransmitter in granule cells and CA3 pyramidal cells. Importantly, these alterations are long lasting in that they can be detected as long as one month after the last kindled seizure. This persistence implicates them in playing a role in the expression of the hyperexcitability of the kindled brain. What remain unclear are the molecular mechanisms underlying the expression of these novel receptor populations.
An early leading hypothesis for the molecular basis of NMDARK was that NMDARK arose from a kindling-induced modulation of receptor subunit composition. This hypothesis arose from the results of studies of recombinant NMDA receptors composed of cloned NMDA receptor subunits. As described above, such studies indicated that subunit composition plays an important role in the determination of receptor properties. Subunit composition is clearly important also in the determination of NMDA receptor antagonist binding sites—since heteromeric receptors consisting of at least NMDAR1 and NR2A are necessary for the production of both competitive and uncompetitive NMDA receptor antagonist binding sites.124 Receptors composed of NMDAR1 or NR2A alone fail to recognize NMDA receptor antagonists. Such results suggest that it is the three-dimensional structure created by heterologous subunit interactions that is important in the determination of antagonist binding, and that expression of different combinations of heterologous subunits could create antagonist-binding sites with novel properties.
A series of studies was designed to test the hypothesis that altered subunit composition results in NMDARK. Subunit composition of a neurotransmitter receptor can be regulated in several ways, including levels of subunit gene expression; processing of heterogeneous nuclear RNA (hnRNA) as in alternative splicing; and post-transcriptional mechanisms (i.e., the regulation of absolute protein levels of subunits). Experiments have been done to test all of these possibilities.
A study measuring the levels of mRNA expression of NMDA receptor subunit genes in the hippocampus of control and kindled animals did not find any difference in the expression of NMDAR1, NR2A, NR2B, NR2C, or NR2D either one day or one month after the last kindled seizure.96 Follow-up experiments examined the expression of specific splice isoforms of NMDAR1 in hippocampal subregions (i.e., mRNA transcripts arising from the alternative splicing of hnRNA of NMDAR1). These studies revealed an interesting short-term regulation of a specific splice cassette of NMDAR1 but failed to demonstrate any differences between control and kindled animals one month after the last kindled seizure.125,126 The lack of regulation of alternative splicing one month after the last kindled seizure indicates that differential expression of splice isoforms does not underlie the expression of NMDARK. The failure to detect a difference at the mRNA level suggested that protein levels of NMDA receptor subunits might be regulated independently of transcript levels. Therefore, a study measuring NMDA receptor protein subunits in discrete hippocampal regions was undertaken. In this study, the levels of NMDAR1, NR2A, and NR2B were measured in fascia dentata, CA3, and CA1 of the hippocampus of control and kindled animals one month after the last kindled seizure. These NMDA receptor subunit proteins were measured because their mRNA is expressed in the hippocampus of adult control and kindled animals (as opposed to NR2C or NR2D, which show very little or no expression). No differences in protein expression were detected.127
The above findings, taken together, suggest that an alteration in subunit composition of the NMDA receptor (as a result of differential subunit mRNA or protein expression) is not likely responsible for the expression of NMDARK in either dentate granule or CA3 pyramidal cells.
The Phosphorylation Hypothesis
The inability to detect changes in the subunit composition of the NMDA receptor in the kindling model has led to an alternative hypothesis that has been only partly tested. This hypothesis states that kindling induces an alteration in the levels of phosphorylation of NMDA receptor subunits.127,128 This idea stems from the observations that NMDA receptor subunits can be phosphorylated,129,130 that phosphorylation of NMDA receptors can lead to alterations (i.e., an enhancement) in receptor function,131–136 and that phosphorylation cascades might be altered in kindling.116,137–139 The importance of the regulation of NMDA receptor responses by phosphorylation is further indicated by the recent discovery that yotiao, an NMDA receptor-associated protein, binds both a phosphatase (dephosphorylating enzyme) and a kinase (phosphorylating enzyme).140 These enzymes were found to regulate channel activity, with phosphatase activity limiting channel responses and kinase activity enhancing channel responses.140 In regard to kindling, the concept is that phosphorylation of the NMDA receptor would result in a conformational change leading to a receptor displaying the properties of NMDARK. To date, direct study of phosphorylation of NMDA receptor subunits has been done only for phosphorylation of tyrosine residues. When examining NMDA receptor subunit protein isolated from both whole hippocampus and from CA3, no differences in tyrosine phosphorylation were detected between kindled and control animals for NMDAR1, NR2A, or NR2B at one month after the last kindled seizure.128,141 Examination of serine/threonine phosphorylation has not yet been done, but it will represent an important component of future research.
RELEVANCE OF KINDLING TO OTHER SENSITIZATION PHENOMENA
Several points can be derived from the above discussion of the kindling model of epilepsy that may have relevance to sensitization models in general.
Repeated Stimulus and Sensitization
The development of kindled seizures is contingent on the repeated application of a stimulus over time. During kindling, the strength of the repeated stimulus does not vary; however, the behavioral response to the stimulus becomes progressively more severe over time. By analogy, repeated stimuli in psychiatric illness (be it an episode of affective illness, exacerbation of psychosis, environmental or physical stress, or exogenous substances) may over time lead to a progressively more severe clinical presentation. For example, such a kindling-like effect is seen in the increased vulnerability of alcoholics to alcohol withdrawal, manifested in adverse withdrawal effects, in the setting of a prior history of withdrawal from alcohol.142,143 The study of enhanced sensitivity to alcohol withdrawal has been facilitated by the development of animal models, which have validated the idea that repeated stimuli (i.e., episodes of alcohol withdrawal) are required for behavioral sensitivity (i.e., withdrawal seizures).11,144
Permanence of Sensitization
The enhanced susceptibility to behavioral seizures persists for the lifetime of the kindled animal. Once an animal is kindled, it remains hypersensitive to the stimulus, even months to years after the last kindled seizure. One may propose more broadly that once sensitization occurs, it may be permanent. Again, using alcohol withdrawal as an example, experimental evidence suggests that hypersensitivity to withdrawal from alcohol may be an irreversible process.145 This concept of permanent hypersensitivity strengthens the rationale for discerning the essential stimuli for sensitization in psychiatric illness. Knowledge of the stimulus (be it an episode of mania in bipolar disorder, an exacerbation of psychosis in schizophrenia, a hit from the crack cocaine pipe in cocaine addiction, or childhood physical or emotional abuse) could lead to the prevention of repeated exposure, and, possibly, the prevention of permanent hypersensitivity to the stimulus.
NMDA Receptor Mediation and Sensitivity Syndromes
NMDA receptor–mediated responses appear to be important for the development and expression of kindled seizures. The fact that NMDA receptor antagonists inhibit seizures during kindling initiation indicates the importance of NMDA receptor–mediated responses in the development of kindling. The discovery of an enhanced sensitivity of NMDA receptor–mediated responses following the induction of kindling suggests that these receptors play a role in the expression of neuronal hyperexcitability. A lasting enhancement of NMDA receptor–mediated activity may underlie diverse sensitization models, where neuroanatomical locale of the receptor may be the determinant of the expressed behavior.
Such mechanisms have been suggested for the alcohol withdrawal syndrome146,147 as well as for the development of enhanced pain sensation in the “windup” or “central hypersensitivity” model.19–21 Responses to stress offer a remarkable correlation to what has been described in the kindling model. Here, the occurrence of a single social stress experience (in this case, confrontation with a dominant opponent) led to an increase in NMDA receptor competitive antagonist binding restricted to region CA3.148 Conversely, a sensitization process that results in a decreased NMDA receptor function could be important in schizophrenia in light of the NMDA receptor hypofunction hypothesis of schizophrenia (see below). Therefore, observations derived from the kindling model may be broadly applicable to a variety of sensitization syndromes.
NMDA Receptor Function and Schizophrenia
Lasting changes intrinsic to the NMDA receptor occur with kindling. Important pharmacological and functional changes in NMDA receptor–mediated responses occur following kindling. These include a differential recognition of competitive antagonists, an enhanced sensitivity to agonists, increases in mean open time of the channel, decreases in Mg2+ sensitivity, a decreased binding affinity for a competitive antagonist, and a reduced potency of competitive antagonists in inhibiting NMDA receptor function.
An NMDA receptor with these properties would be expected to be more sensitive to endogenous glutamate but less sensitive to a class of antiseizure drugs, the competitive NMDA receptor antagonists. Indeed, NMDA receptor antagonists are only minimally effective in inhibiting seizures in fully kindled animals. One might propose that the reason for this “treatment refractoriness” is that the molecular structure of the NMDA receptor has been altered in such a way as to make it less sensitive to the antiseizure drug. By analogy, neurotransmitter receptor plasticity may underlie the development of treatment refractoriness in psychiatric illness.
Studies to date have revealed that these changes in receptor function in the kindling model are not related to alterations in NMDA receptor subunit gene expression, to differences in alternative splicing of NMDAR1, to changes in the levels of subunit protein, or to a difference in the tyrosine phosphorylation of NMDA receptor subunits. Current hypotheses are focused on post-translational modification of the receptor (e.g., serine/threonine phosphorylation). An understanding of the molecular basis of NMDARK could lead to the development of more efficacious drugs for the treatment of epilepsy as well as for related illnesses characterized by sensitization.
The kindling studies reveal that the NMDA receptor remains plastic in the brains of adult animals, and this plasticity may result in behavioral pathology. This observation may have direct relevance to the NMDA receptor hypofunction model of schizophrenia. This model is derived mainly from observations that NMDA receptor antagonists (such as PCP and ketamine) induce behavioral, perceptual, and cognitive effects similar to schizophrenia when given to normal volunteers.149–152 Likewise, these drugs exacerbate symptoms in schizophrenic patients.153,154 More recently, transgenic mice deficient in the expression of the NMDAR1 subunit showed behavioral changes similar to those seen in pharmacological models of psychosis.155 These behaviors could be ameliorated by the administration of antipsychotic medication.155 These findings indicate that reduced functioning of NMDA receptors, whether through pharmacological or genetic manipulation, produces a syndrome similar to schizophrenia.
A direct consequence of the NMDA receptor hypofunction hypothesis has been the use of glycine-site agonists in schizophrenic patients in an effort to augment NMDA receptor–mediated function. (Recall that glycine is a necessary co-agonist at the NMDA receptor.) Taken together, these studies have found that treatment with glycinergic agents results in a modest improvement in the negative symptoms of schizophrenic patients.156–160 The mechanisms underlying this treatment effect are currently unknown.
The Phosphorylation Hypothesis and Schizophrenia
In light of the results of kindling studies revealing NMDA receptor pharmacological and functional plasticity in the adult brain, one could posit that the putative NMDA receptor hypofunction in schizophrenia results from the induction of altered NMDA receptors in the disease state. These receptors might have a lower affinity for glycine, explaining why administration of exogenous glycine-agonists results in a favorable clinical response. Additionally, one could imagine that these receptors might be less sensitive to glutamate, and, perhaps, more sensitive to Mg2+ block (an area of the open channel close to where PCP acts). The end result would be a receptor that would function poorly under normal conditions. The reader may recognize that this imagined receptor has properties virtually opposite to those of NMDARK. Therefore, an understanding of the molecular basis of NMDARK might have direct relevance to the putative NMDA hypofunction in schizophrenia. A speculative hypothesis based on this idea is that an altered balance between kinase (enzymes that phosphorylate proteins) and phosphatase (enzymes that dephosphorylate proteins) activity occurs in the pathological state (Figure 3). For example, in kindling the receptor might be hyperphosphorylated (enhancing receptor activity), and in schizophrenia the receptor might be hypophosphorylated (diminishing receptor activity). To further speculate, sensitization involving NMDA receptor plasticity may occur during the development of schizophrenia as it does in the development of kindled seizures using similar (but opposing) signal transduction pathways. Attractive candidate proteins that might be involved in the development of kindling and/or schizophrenia include yotiao (a scaffold protein associated with the NMDA receptor), and type I protein phosphatase (PP1) and protein kinase A (PKA), both of which are associated directly with yotiao (and thus with the NMDA receptor), regulating receptor function via phosphorylation state of the receptor.140
OTHER CONSIDERATIONS
The Kindling Model in Animals and Human Pathology
The foregoing discussion of NMDA receptor plasticity in kindling is a synthesis of observations derived from the study of an animal model of epilepsy. Because of the precise neuroanatomical, pharmacological, and molecular biological techniques employed in these studies, analogous studies in humans, at present, cannot be undertaken. The utility of any animal model is determined by how well it recapitulates the human disease, and, on these terms, kindling may be considered a useful model of complex partial epilepsy. Kindling is analogous to complex partial epilepsy in that similar EEG activity is recorded from intracerebral electrodes during seizures, similar behaviors are observed, and kindled seizures and complex partial epilepsy have similar sensitivities to conventional antiseizure drugs.24 Additionally, alterations in glutamatergic function, including NMDA receptor function, have been identified in studies of human tissue excised from the brains of epileptic patients treated surgically for refractory epilepsy. These include the presence of an NMDA receptor–mediated component in the perforant path EPSP in dentate granule cells of human epileptics161 and the identification of an increased NMDA receptor apparent density in hippocampal tissue from epileptic patients.162 Finally, some antiseizure drugs have been shown to have NMDA receptor antagonist activity. The strongest case to be made in this regard is the drug felbamate. Felbamate is effective in the treatment of patients with poorly controlled partial and secondarily generalized seizures.163 The drug, in addition to its GABAergic properties, has been demonstrated to act as an NMDA receptor channel blocker164,165 and an antagonist of the NMDA receptor at the glycine site.166 Taken together, the correlative observations from the animal model and the human disease, the findings from investigations of tissue derived from the brains of epileptic patients, and the mechanisms of novel antiseizure drugs all strengthen the argument that alterations in NMDA receptor function as identified in the kindling model have direct relevance to human pathology.
Stages of Sensitization and Modes of Therapeutic Intervention
The discussion above focused mainly on mechanisms underlying the expression of hyperexcitability in the kindling model (i.e., the induction of NMDARK leading to enhanced glutamatergic function). It is important to understand that in any sensitization process, there are at least three stages: development, expression, and persistence (or maintenance). An understanding of the molecular mechanisms involved in the development of pathologic hypersensitivity may lead to practical approaches designed to prevent the disease in high-risk patients (e.g., severe head injury patients in epilepsy or individuals who have experienced severe psychological/physical trauma in posttraumatic stress disorder). The understanding of the cellular and molecular mechanisms involved in the expression of hypersensitivity relates to the elucidation of the particular molecules (e.g., receptors, kinases, phosphatases) and mediators (e.g., Ca2+, adenosine 3′,5′-cyclic monophosphate [cAMP]) whose modulation and/or activation may result in abnormal neuronal activity. Insight into the pathophysiology of the expression of sensitization in molecular terms may lead to more selective and specific treatments designed to suppress abnormal activity in the brains of affected patients. Lastly, an understanding of the persistence of sensitization addresses the molecular, cellular, and structural processes that sustain the pathologic response of the “sensitized” brain. Insight into the mechanisms of the persistence of sensitization may eventually lead to cures for neuropsychiatric conditions that develop as a result of sensitization processes. The distinctions among these stages are important, although they all may reside on a continuum of molecular, cellular, and anatomical modifications that ultimately lead to the “sensitized” state. Alternatively, each stage of sensitization may have distinct molecular and cellular substrates, necessitating unique therapeutic interventions for each aspect of disease progression.
Other Processes Affecting Kindling and Sensitization Phenomena
The NMDA receptor represents but one target of plasticity that has been identified in the brains of kindled animals. A discussion of this target was chosen because of its importance in the mediation of glutamatergic excitability, its attractiveness as a therapeutic substrate in neuropsychiatric disease, its role in neuronal plasticity in development and maturity, its putative role in psychiatric illness (especially schizophrenia), and its importance in other, nonkindling sensitization processes. In addition to molecular plasticity of NMDA receptors, there are kindling-induced neuroanatomical alterations (sprouting), changes in gene expression, differential growth factor expression, and alterations in other neurotransmitter systems.167–172 The relative contribution of each of these to the overall “kindled” state is currently unknown, although experiments using transgenic animals are beginning to clarify the issue.173–177 Likewise, the development of sensitization probably arises from the interactions of a variety of molecular, cellular, and structural alterations.
Finally, postsynaptic NMDA receptors represent but one area where long-lasting functional changes may have relevance to sensitization. Sensitization, depending on the stage of progression, may be manifested on a cellular/molecular level by 1) presynaptic changes (e.g., enhanced neurotransmitter release, diminished/altered expression of autoreceptors, differential gene expression in presynaptic neurons); 2) postsynaptic changes (e.g., receptor plasticity, altered second messenger systems, differential gene expression in postsynaptic neurons); and/or 3) neural network changes (e.g., alterations in inhibitory control between brain regions, unmasking or strengthening of connections between brain regions, neurodegenerative changes “short-circuiting” neuronal connectivity, initiation of novel connectivity via sprouting mechanisms). It is simplistic to posit that one process predominates in sensitization phenomena, especially when different mechanisms may achieve similar phenotypic results or end-target modifications.
For example, although it is proposed that NMDA receptor hypofunction in schizophrenia and hyperfunction in kindling might have a common molecular substrate (phosphorylation state of the NMDA receptor; see Figure 3), the chains of events leading to these NMDA receptor alterations might be very different. These differences might include relative weight of initiating neurotransmitter activities (e.g., dopamine vs. glutamate), cell populations affected, pattern of gene expression, specific phosphatases and kinases involved, and overall network strengthening/weakening. Regardless of these potential differences, the knowledge of the molecular processes leading to NMDA receptor hyperfunction in kindling would be of great utility in the generation of testable hypotheses for the NMDA receptor hypofunction model of schizophrenia (as well as other psychiatric diseases conceptualized as sensitization processes).
CONCLUSION
The kindling model of epilepsy stands as the prototypical model of sensitization. Because sensitization has been proposed as a pathophysiological mechanism in a number of psychiatric illnesses, a basic understanding of the kindling model of epilepsy is essential. Likewise, knowledge of the molecular basis of kindling-induced hyperexcitability may have broader applicability to our understanding of other sensitization phenomena. In particular, an understanding of the molecular mechanisms underlying enhanced NMDA receptor function following kindling might have direct relevance to the NMDA receptor hypofunction hypothesis of schizophrenia. The clinical goals allied with the search for a molecular understanding of sensitization are the rational design of more efficacious drugs and, perhaps, the prevention of more severe illness.
ACKNOWLEDGMENTS
The author thanks Drs. James O. McNamara, Jeffrey A. Lieberman, and Diana O. Perkins for their support and encouragement over the years.
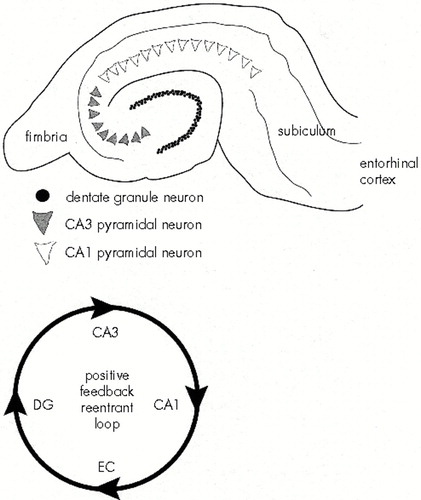
FIGURE 1. Schematized drawing of a hippocampal slice and the hippocampal circuitry (the positive feedback reentrant loop)Top: the major cell populations of the hippocampus—the dentate granule cells of the dentate gyrus, the CA3 pyramidal cells, and the CA1 pyramidal cells. Bottom: one of several impulse pathways through the hippocampus. Axons of entorhinal cortical (EC) neurons contact dentate granule cells (DG) via the perforant path. The axons of the granule cells (the mossy fibers) terminate primarily on the apical dendrites of the CA3 pyramidal cells. The CA3 pyramidal cells send Schaffer collaterals to the apical dendrites of the CA1 pyramidal cells. A significant percentage of axons from CA1 terminate in the subiculum. In turn, neurons in the subiculum project to the entorhinal cortex, thus closing the circuit passing from entorhinal cortex to hippocampus back to the entorhinal area. All connections in this feedback loop are excitatory (glutamatergic).
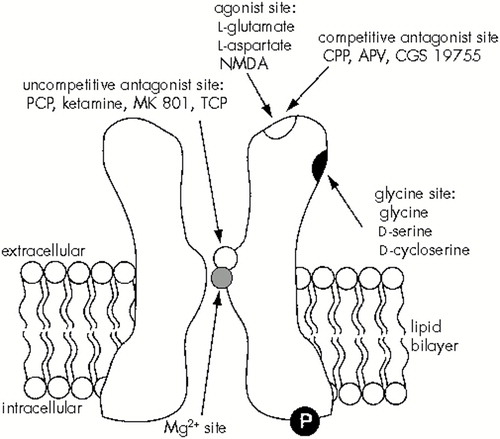
FIGURE 2. Schematic of the N-methyl-d-aspartate (NMDA) subtype of glutamate receptor, illustrating some of the regulatory domains discussed in the textFor the channel to open (thus allowing the flow of calcium, sodium, and potassium), two events must occur simultaneously: 1) glutamate and glycine must bind to their sites, and 2) the cell must be depolarized (i.e., attain a more positive membrane potential) such that magnesium is released from its site within the channel pore. The “P” in the figure indicates that the receptor may be regulated by phosphorylation of the intracellular domains. Probably four subunits come together to form the receptor channel complex. At least one of those subunits must be the subunit NMDAR1. Members of the NR2 family contribute subunits as well, helping to determine channel functional properties. APV=2-amino-5-phosphonovalerate; CGS 19755=1-(cis-2-carboxypiperidine-4-yl)-propyl-1-phosphonic acid; CPP=3-(2-carboxypiperazin-4-yl)-propyl-1-phosphonic acid; MK 801=dizocilpine; PCP=phencyclidine; TCP=1-(1-(2-thienyl)-cyclohexyl)-piperidine.
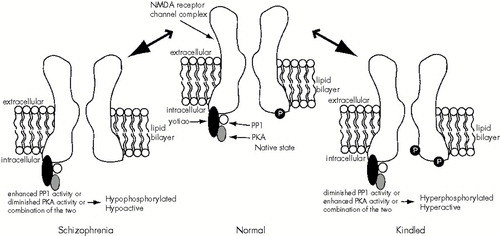
FIGURE 3. A speculative model by which opposing processes occurring during sensitization could lead to NMDA receptors' displaying enhanced activity (as in the dentate granule and CA3 neurons of the hippocampus of kindled animals) or diminished activity (as in the NMDA receptor hypofunction hypothesis of schizophrenia)Here, the relative activities of kinases (phosphorylating enzymes) and phosphatases (dephosphorylating enzymes) determine the overall phosphorylation state of the receptor (and hence its activity). Evidence suggests that phosphorylation of the receptor results in increased NMDA receptor activity, whereas dephosphorylation results in decreased activity. In the model, yotiao is a scaffolding protein that associates with the NMDA receptor. Yotiao also binds type 1 protein phosphatase (PP1) and adenosine 3′,5′-cyclic monophosphate (cAMP)-dependent protein kinase (PKA), bringing these enzymes in close proximity to the receptor. The close apposition of these enzymes to the NMDA receptor suggests a tight correlation of their activity with ion flow (or lack thereof) through the NMDA receptor. However, many kinases and phosphatases (including tyrosine kinases, protein kinase C, and calcium-sensitive kinase and phosphatase) are able to interact with the NMDA receptor, and these may be important in the model depicted. Likewise, the model suggests increased/decreased phosphorylation state of individual receptors (e.g., phosphate groups on two sites rather than one or none), but an equally plausible possibility is the addition or removal of a phosphate group from a single specific site in a subpopulation of NMDA receptors.
1 Post RM, Weiss SR: A speculative model of affective illness cyclicity based on patterns of drug tolerance observed in amygdala-kindled seizures. Mol Neurobiol 1996; 13:33–60Crossref, Medline, Google Scholar
2 Stoll AL, Severus WE: Mood stabilizers: shared mechanisms of action at postsynaptic signal-transduction and kindling processes. Harv Rev Psychiatry 1996; 4:77–89Crossref, Medline, Google Scholar
3 Post RM, Denicoff KD, Frye MA, et al: A history of the use of anticonvulsants as mood stabilizers in the last two decades of the 20th century. Neuropsychobiology 1998; 38:152–166Crossref, Medline, Google Scholar
4 Ghaemi SN, Boiman EE, Goodwin FK: Kindling and second messengers: an approach to the neurobiology of recurrence in bipolar disorder. Biol Psychiatry 1999; 45:137–144Crossref, Medline, Google Scholar
5 Post RM, Weiss SR: Neurobiological models of recurrence in mood disorder, in Neurobiology of Mental Illness, edited by Charney DS, Nestler EJ, Bunney BS. New York, Oxford University Press, 1999, pp 365–384Google Scholar
6 Lieberman JA, Kinon BJ, Loebel AD: Dopaminergic mechanisms in idiopathic and drug-induced psychoses. Schizophr Bull 1990; 16:97–110Crossref, Medline, Google Scholar
7 Lieberman JA, Sheitman BB, Kinon BJ: Neurochemical sensitization in the pathophysiology of schizophrenia: deficits and dysfunction in neuronal regulation and plasticity. Neuropsychopharmacology 1997; 17:205–229Crossref, Medline, Google Scholar
8 Sheitman BB, Lieberman JA: The natural history and pathophysiology of treatment-resistant schizophrenia. J Psychiatr Res 1998; 32:143–150Crossref, Medline, Google Scholar
9 Duncan GE, Sheitman BB, Lieberman JA: An integrated view of pathophysiological models of schizophrenia. Brain Res Rev 1999; 29:250–264Crossref, Medline, Google Scholar
10 Davis WM: Psychopharmacologic violence associated with cocaine abuse: kindling of a limbic dyscontrol syndrome? Prog Neuropsychopharmacol Biol Psychiatry 1996; 20:1273–1300Google Scholar
11 Becker HC: The alcohol withdrawal “kindling” phenomenon: clinical and experimental findings. Alcohol Clin Exp Res 1996; 20(8, suppl):121A–124AGoogle Scholar
12 Phillips TJ, Roberts AJ, Lessov CN: Behavioral sensitization to alcohol: genetics and the effects of stress. Pharmacol Biochem Behav 1997; 57:487–493Crossref, Medline, Google Scholar
13 Kalivas PW, Pierce RC, Cornish J, et al: A role for sensitization in craving and relapse in cocaine addiction. J Psychopharmacol 1998; 12:49–53Crossref, Medline, Google Scholar
14 Singleton EG, Gorelick DA: Mechanisms of alcohol craving and their clinical implications. Recent Dev Alcohol 1998; 14:177–195Medline, Google Scholar
15 Grillon C, Southwick SM, Charney DS: The psychobiological basis of posttraumatic stress disorder. Mol Psychiatry 1996; 1:278–297Medline, Google Scholar
16 Post RM, Weiss SR, Smith M, et al: Kindling versus quenching: implications for the evolution and treatment of posttraumatic stress disorder. Ann NY Acad Sci 1997; 821:285–295Crossref, Medline, Google Scholar
17 Bell IR: White paper: neuropsychiatric aspects of sensitivity to low-level chemicals: a neural sensitization model. Toxicol Ind Health 1994; 10:277–312Medline, Google Scholar
18 Bell IR, Rossi J III, Gilbert ME, et al: Testing the neural sensitization and kindling hypothesis for illness from low levels of environmental chemicals. Environ Health Perspect 1997; 105(2, suppl):539–547Google Scholar
19 Dougherty PM, Willis WD: Enhancement of spinothalamic neuron responses to chemical and mechanical stimuli following combined micro-iontophoretic application of N-methyl-d-aspartic acid and substance P. Pain 1991; 47:85–93Crossref, Medline, Google Scholar
20 Coderre TJ, Melzack R: The role of NMDA receptor-operated calcium channels in persistent nociception after formalin-induced tissue injury. J Neurosci 1992; 12:3671–3675Google Scholar
21 Stevens CF: No pain, no gain. Curr Opin Neurobiol 1992; 9:497–499Google Scholar
22 Goddard GV, McIntyre EC, Leech CK: A permanent change in brain function resulting from daily electrical stimulation. Exp Neurol 1969; 25:295–330Crossref, Medline, Google Scholar
23 McNamara JO, Morrisett R, Nadler JV: Recent advances in understanding mechanisms of the kindling model. Adv Neurol 1992; 57:555–560Medline, Google Scholar
24 McNamara JO, Bonhaus DW, Shin C: The kindling model of epilepsy, in Epilepsy: Models, Mechanisms, and Concepts, edited by Schwartzkroin PA. New York, Cambridge University Press, 1993, pp 27–47Google Scholar
25 McNamara JO: Kindling model of epilepsy. Adv Neurol 1986; 44:303–318Medline, Google Scholar
26 Goddard GV: Development of epileptic seizures through brain stimulation at low intensity. Nature 1967; 214:1020–1021Google Scholar
27 Racine RJ: Modulation of seizure activity by electrical stimulation, II: motor seizure. Electroencephalogr Clin Neurophysiol 1972; 32:281–294Crossref, Medline, Google Scholar
28 Dichter MA: The epilepsies and convulsive disorders, in Harrison's Principles of Internal Medicine, edited by Wilson JD, Braunwald E, Isselbacher KJ, et al. New York, McGraw-Hill, 1991, pp 1968–1976Google Scholar
29 Swanson TH: The pathophysiology of human mesial temporal lobe epilepsy. J Clin Neurophysiol 1995; 12:2–22Crossref, Medline, Google Scholar
30 Kraus JE, McNamara JO: Measurement of cortical neurotransmitters with radioligand binding: insights into the mechanisms of kindling-induced epilepsy, in The Cortical Neuron, edited by Gutnick MJ, Mody I. New York, Oxford University Press, 1995, pp 264–275Google Scholar
31 Byrne MC, Gottlieb R, McNamara JO: Amygdala kindling induces muscarinic cholinergic receptor declines in a highly specific distribution within the limbic system. Exp Neurol 1980; 69:85–98Crossref, Medline, Google Scholar
32 McNamara JO, Peper AM, Patrone V: Repeated seizures induce long-term increase in hippocampal benzodiazepine receptors. Proc Natl Acad Sci USA 1980; 77:3029–3032Google Scholar
33 Valdes F, Dasheiff RM, Birmingham F, et al: Benzodiazepine receptor increases after repeated seizures: evidence for localization to dentate granule cells. Proc Natl Acad Sci USA 1982; 79:193–197Crossref, Medline, Google Scholar
34 Savage DD, Dashieff RM, McNamara JO: Kindled seizure-induced reduction of muscarinic cholinergic receptors in rat hippocampal formation: evidence for localization to dentate granule cells. J Comp Neurol 1983; 221:106–112Crossref, Medline, Google Scholar
35 Dasheiff RM, McNamara JO: Intradentate colchicine retards the development of amygdala kindling. Ann Neurol 1982; 11:347–352Crossref, Medline, Google Scholar
36 Frush DP, Giacchino JL, McNamara JO: Evidence implicating dentate granule cells in development of entorhinal kindling. Exp Neurol 1986; 92:92–101Crossref, Medline, Google Scholar
37 Savage DD, Rigsbee LC, McNamara JO: Knife cuts of the entorhinal cortex: effects on development of amygdaloid kindling and seizure-induced decrease of muscarinic cholinergic receptors. J Neurosci 1985; 5:408–413Crossref, Medline, Google Scholar
38 King GL, Dingledine R, Giacchino JL, et al: Abnormal neuronal excitability in hippocampal slices from kindled rats. J Neurophysiol 1985; 54:1295–1304Google Scholar
39 Curtis DR, Phillis JW, Watkins JC: Chemical excitation of spinal neurones. Nature 1959; 183:611–612Crossref, Medline, Google Scholar
40 Curtis DR, Watkins JC: The excitation and depression of spinal neurones by structurally related amino acids. J Neurochem 1960; 6:117–141Crossref, Medline, Google Scholar
41 Duggan AW: The differential sensitivity to l-glutamate and l-aspartate of spinal interneurones and Renshaw cells. Exp Brain Res 1974; 19:522–528Crossref, Medline, Google Scholar
42 Johnston GAR, Curtis DR, Davies J, et al: Spinal interneuron excitation by conformationally restricted analogues of l-glutamic acid. Nature 1974; 248:804–805Crossref, Medline, Google Scholar
43 Fagg GE, Massieu L: Excitatory amino acid subtypes, in Excitatory Amino Acid Antagonists, edited by Meldrum BS. Boston, Blackwell Scientific, 1991, pp 39–63Google Scholar
44 Davies J, Watkins JC: Effects of magnesium ions on the responses of spinal neurones to excitatory amino acids and acetylcholine. Brain Res 1977; 130:364–368Crossref, Medline, Google Scholar
45 Biscoe TJ, Davies J, Dray A, et al: Depression of synaptic excitation and of amino acid-induced excitatory responses of spinal neurones by d-α-aminoadipate, α,ε-diaminopimelic acid and HA-966. Eur J Pharmacol 1977; 45:315–316Crossref, Medline, Google Scholar
46 Davies J, Francis AA, Jones AW, et al:2-amino-5-phosphonovalerate (2APV), a potent and selective antagonist of amino acid-induced and synaptic excitation. Neurosci Lett 1981; 21:77–81Google Scholar
47 Davies J, Evans RH, Herrling PL, et al: CPP, a new potent and selective NMDA antagonist. Depression of central neuron responses, affinity of [3H]d-APV binding sites on brain membranes and anticonvulsant activity. Brain Res 1986; 382:169–173Crossref, Medline, Google Scholar
48 Watkins JC: Structure/activity relations of competitive NMDA receptor antagonists, in Excitatory Amino Acid Antagonists, edited by Meldrum BS. Boston, Blackwell Scientific, 1991, pp 84–100Google Scholar
49 Meldrum BS: Historical introduction: key discoveries, in Excitatory Amino Acid Antagonists, edited by Meldrum BS. Boston, Blackwell Scientific, 1991, pp 1–13Google Scholar
50 Johnson JW, Ascher P: Glycine potentiates the NMDA response in cultured mouse brain neurons. Nature 1987; 325:529–531Crossref, Medline, Google Scholar
51 Kleckner NW, Dingledine R: Requirement for glycine in activation of NMDA receptors expressed in Xenopus oocytes. Science 1988; 238:355–358Google Scholar
52 Leeson PD, Iversen LL: The glycine site on the NMDA receptor: structure-activity relationships and therapeutic potential. J Med Chem 1994; 37:4053–4067Google Scholar
53 Anis NA, Berry SC, Burton NR, et al: The dissociative anesthetics, ketamine and phencyclidine, selectively reduce excitation of central mammalian neurons by N-methylaspartate. Br J Pharmacol 1983; 79:565–575Crossref, Medline, Google Scholar
54 Wong EHF, Kemp JA, Priestly T, et al: The anticonvulsant MK 801 is a potent N-methyl-d-aspartate antagonist. Proc Natl Acad Sci USA 1986; 83:7104–7108Google Scholar
55 Ransom RW, Stec NL: Cooperative modulation of [3H]MK 801 binding to the N-methyl-d-aspartate receptor-ion channel complex by l-glutamate, glycine, and polyamines. J Neurochem 1988; 51:830–836Crossref, Medline, Google Scholar
56 Williams K, Romano C, Molinoff PB: Effects of polyamines on the binding of [3H]MK 801 to the NMDA receptor: pharmacological evidence for the existence of a polyamine recognition site. Mol Pharmacol 1989; 36:575–581Medline, Google Scholar
57 Rock DM, Macdonald RL: The polyamine spermine has multiple actions on N-methyl-d-aspartate receptor single-channel currents in cultured cortical neurons. Mol Pharmacol 1992; 41:83–88Medline, Google Scholar
58 Benveniste M, Mayer ML: Multiple effects of spermine on N-methyl-d-aspartic acid receptor responses of rat cultured hippocampal neurones. J Physiol 1993; 464:131–163Crossref, Medline, Google Scholar
59 Westbrook GL, Mayer ML: Micromolar concentrations of Zn2+ antagonize NMDA and GABA responses of hippocampal neurons. Nature 1987; 328:640–643Crossref, Medline, Google Scholar
60 Peters S, Koh J, Choi DW: Zinc selectively blocks the action of N-methyl-d-aspartate on cortical neurons. Science 1987; 236:589–593Crossref, Medline, Google Scholar
61 Yeh G-C, Bonhaus DW, McNamara JO: Evidence that zinc inhibits N-methyl-d-aspartate receptor-gated ion channel activation by noncompetitive antagonism of glycine binding. Mol Pharmacol 1990; 38:14–19Medline, Google Scholar
62 Aizenman E, Lipton SA, Loring RH: Selective modulation of NMDA responses by reduction and oxidation. Neuron 1989; 2:1257–1263Google Scholar
63 Lipton SA: Prospects for clinically tolerated NMDA antagonists: open-channel blockers and alternative redox states of nitric oxide. Trends Neurosci 1993; 16:527–532Crossref, Medline, Google Scholar
64 Paoletti P, Ascher P: Mechanosensitivity of NMDA receptors in cultured mouse central neurons. Neuron 1994; 13:645–655Crossref, Medline, Google Scholar
65 MacDermott AB, Mayer ML, Westbrook GL, et al: NMDA receptor activation increases cytoplasmic calcium concentration in cultured spinal cord neurons. Nature 1986; 321:519–522Crossref, Medline, Google Scholar
66 Mayer ML, Westbrook GL: Permeation and block of N-methyl-d-aspartic acid receptor channels by divalent cations in mouse cultured central neurons. J Physiol 1987; 394:501–527Crossref, Medline, Google Scholar
67 Ascher P, Nowak L: The role of divalent cations in the N-methyl-d-aspartate responses of mouse central neurons in culture. J Physiol 1988; 399:247–266Crossref, Medline, Google Scholar
68 Nowak L, Bregestovski P, Ascher P, et al: Magnesium gates glutamate-activated channels in mouse central neurons. Nature 1984; 307:462–465Crossref, Medline, Google Scholar
69 Jahr CE, Stevens CF: A quantitative description of NMDA receptor channel kinetic behaviour. J Neurosci 1990; 10:1830–1837Google Scholar
70 Dingledine R: NMDA receptors: what do they do? Trends Neurosci 1986; 9:47–49Google Scholar
71 Hollmann M, O'Shea-Greenfield A, Rogers SW, et al: Cloning by functional expression of a member of the glutamate receptor family. Nature 1989; 342:643–648Crossref, Medline, Google Scholar
72 Boulter J, Hollmann M, O'Shea-Greenfield A, et al: Molecular cloning and functional expression of glutamate receptor subunit genes. Science 1990; 249:1033–1037Google Scholar
73 Keinänen K, Wisden W, Sommer B, et al: A family of AMPA selective glutamate receptors. Science 1990; 249:556–560Crossref, Medline, Google Scholar
74 Nakanishi N, Shneider NA, Axel R: A family of glutamate receptor genes: evidence for the formation of heteromultimeric receptors with distinct channel properties. Neuron 1990; 5:569–581Crossref, Medline, Google Scholar
75 Bettler B, Boulter J, Hermans-Borgmeyer I, et al: Cloning of a novel glutamate receptor subunit GluR5: expression in nervous system during development. Neuron 1990; 5:583–595Crossref, Medline, Google Scholar
76 Egebjerg J, Bettler B, Hermans-Borgmeyer I, et al: Cloning of a cDNA for a glutamate receptor subunit activated by kainate but not AMPA. Nature 1991; 351:745–748Crossref, Medline, Google Scholar
77 Werner P, Voight M, Keinänen K, et al: Cloning of a putative high-affinity kainate receptor expressed predominantly in hippocampal CA3 cells. Nature 1991; 351:742–744Crossref, Medline, Google Scholar
78 Bettler B, Egebjerg J, Sharma G, et al: Cloning of a putative glutamate receptor: a low affinity kainate-binding subunit. Neuron 1992; 8:1–20Crossref, Google Scholar
79 Herb A, Burnashev N, Werner P, et al: The KA2 subunit of excitatory amino acid receptors shows widespread expression in brain and forms ion channels with distantly related subunits. Neuron 1992; 8:775–785Crossref, Medline, Google Scholar
80 Sommer B, Burnashev N, Verdoorn TA, et al: A glutamate receptor channel with high affinity for domoate and kainate. EMBO J 1992; 11:1651–1656Google Scholar
81 Moriyoshi K, Masu M, Ishii T, et al: Molecular cloning and characterization of the rat NMDA receptor. Nature 1991; 354:31–37Crossref, Medline, Google Scholar
82 Yamazaki M, Mori H, Araki K, et al: Cloning, expression and modulation of a mouse NMDA receptor subunit. FEBS Lett 1992; 300:39–45Crossref, Medline, Google Scholar
83 Meguro H, Mori H, Araki K, et al: Functional characterization of a heteromeric NMDA receptor channel expressed from cloned cDNAs. Nature 1992; 357:70–74Crossref, Medline, Google Scholar
84 Monyer H, Sprengel R, Schoepfer R, et al: Heteromeric NMDA receptors: molecular and functional distinction of subtypes. Science 1992; 256:1217–1221Google Scholar
85 Ikeda K, Nagasawa M, Mori H, et al: Cloning and expression of the e4 subunit of the NMDA receptor channel. FEBS Lett 1992; 313:34–38Crossref, Medline, Google Scholar
86 Kutsuwada T, Kashiwabuchi N, Mori H, et al: Molecular diversity of the NMDA receptor channel. Nature 1992; 358:36–41Crossref, Medline, Google Scholar
87 Ishii T, Moriyoshi K, Sugihara H, et al: Molecular characterization of the family of the N-methyl-d-aspartate receptor subunits. J Biol Chem 1993; 268:2836–2843Google Scholar
88 Dingledine R: New wave of non-NMDA excitatory amino acid receptors. Trends Pharmacol Sci 1991; 12:360–362Crossref, Medline, Google Scholar
89 Nakanishi S: Molecular diversity of glutamate receptors and implications for brain function. Science 1992; 258:597–603Crossref, Medline, Google Scholar
90 Sommer B, Keinänen K, Verdoorn TA, et al: Flip and flop; a cell-specific functional switch in glutamate-operated channels of the CNS. Science 1990; 249:1580–1585Google Scholar
91 Sugihara H, Moriyoshi K, Ishii T, et al: Structures and properties of seven genes of the NMDA receptor generated by alternative splicing. Biochem Biophys Res Commun 1992; 185:826–836Crossref, Medline, Google Scholar
92 Nakanishi N, Axel A, Shneider NA: Alternative splicing generates functionally distinct N-methyl-d-aspartate receptors. Proc Natl Acad Sci USA 1992; 89:8552–8556Google Scholar
93 Sommer B, Köhler M, Sprengel R, et al: RNA editing in brain controls a determinant of ion flow in glutamate-gated channels. Cell 1991; 67:11–19Crossref, Medline, Google Scholar
94 Seeburg PH: The role of RNA editing in controlling glutamate receptor channel properties. J Neurochem 1996; 66:1–5Crossref, Medline, Google Scholar
95 Rosenmund C, Stern-Bach Y, Stevens CF: The tetrameric structure of a glutamate receptor channel. Science 1998; 280:1596–1599Google Scholar
96 Kraus JE, Yeh G-C, Bonhaus DW, et al: Kindling induces the long-lasting expression of a novel population of NMDA receptors in hippocampal region CA3. J Neurosci 1994; 14:4196–4205Google Scholar
97 Herron CE, Lester RAJ, Coan EJ, et al: Frequency-dependent involvement of NMDA receptors in the hippocampus: a novel synaptic mechanism. Nature 1986; 322:265–268Crossref, Medline, Google Scholar
98 Collingridge GL, Herron CE, Lester RA: Frequency-dependent N-methyl-d-aspartate receptor-mediated synaptic transmission in rat hippocampus. J Physiol 1988; 399:301–312Crossref, Medline, Google Scholar
99 Dingledine R, McBain CJ, McNamara JO: Excitatory amino acid receptors in epilepsy. Trends Pharmacol Sci 1990; 11:334–338Crossref, Medline, Google Scholar
100 Croucher MJ, Collins JF, Meldrum BS: Anticonvulsant action of excitatory amino acid antagonists. Science 1982; 216:899–901Crossref, Medline, Google Scholar
101 Czuczwar SJ, Meldrum BS: Protection against chemically induced seizures by 2-amino-7-phosphonoheptanoic acid. Eur J Pharmacol 1982; 83:335–338Crossref, Medline, Google Scholar
102 Clineschmidt BV, Martin GE, Bunting PR: Anticonvulsant activity of (+)-5-methyl-10,11-dihydro-5H-dibenzo[a,d]cyclohepten-5,10-imine (MK 801): a substance with potent anticonvulsant, central sympathomimetic, and apparent anxiolytic properties. Drug Dev Res 1982; 2:123–134Crossref, Google Scholar
103 McNamara JO, Russel RD, Rigsbee L, et al: Anticonvulsant and anti-epileptogenic actions of MK-801 in the kindling and electroshock models. Neuropharmacology 1988; 27:563–568Crossref, Medline, Google Scholar
104 Mitrovic N, Besson M-J, Maurin Y: Anticonvulsant effects of antagonists of the N-methyl-d-aspartate receptor complex in a genetic model of epilepsy: the quaking mouse. Eur J Pharmacol 1990; 176:357–361Crossref, Medline, Google Scholar
105 Patel S, Chapman AG, Graham JL, et al: Anticonvulsant activity of the NMDA antagonists, d(–)4-(3-phosphonopropyl) piperazine-2-carboxylic acid (d-CPP) and d(–)(ε)-4-(3-phosphonoprop-2-enyl) piperazine-2-carboxylic acid (d-CPPene) in a rodent and a primate model of reflex epilepsy. Epilepsy Res 1990; 7:3–10Crossref, Medline, Google Scholar
106 Mitrovic N, Caboche J, Carré JB, et al: The quaking mouse: an epileptic mutant with alterations affecting the modulatory mechanisms of the NMDA receptor complex. Brain Res 1991; 566:248–254Crossref, Medline, Google Scholar
107 Chapman AG, Graham JL, Patel S, et al: Anticonvulsant activity of two orally active competitive N-methyl-d-aspartate antagonists, CGP 37849 and CGP 39551, against sound-induced seizures in DBA/2 mice and photically induced myoclonus in Papio papio. Epilepsia 1991; 32:578–587Crossref, Medline, Google Scholar
108 De Sarro GB, De Sarro A: Anticonvulsant properties of non-competitive antagonists of the N-methyl-d-aspartate receptor in genetically epilepsy-prone rats: comparison with CPPene. Neuropharmacology 1993; 32:51–58Crossref, Medline, Google Scholar
109 Callaghan DA, Schwark WS: Pharmacological modification of amygdaloid-kindled seizures. Neuropharmacology 1980; 19:1131–1136Google Scholar
110 Peterson DW, Collins JF, Bradford HF: Anticonvulsant action of amino acid antagonists against kindled hippocampal seizures. Brain Res 1984; 311:176–180Crossref, Medline, Google Scholar
111 Löscher W, Nolting B, Hönack D: Evaluation of CPP, a selective NMDA antagonist, in various rodent models of epilepsy: comparison with other NMDA antagonists, and with diazepam and phenobarbital. Eur J Pharmacol 1988; 152:9–17Crossref, Medline, Google Scholar
112 Löscher W, Hönack D: Anticonvulsant and behavioral effects of two novel competitive N-methyl-d-aspartic acid receptor antagonists, CGP 37849 and CGP 39551, in the kindling model of epilepsy: comparison with MK-801 and carbamazepine. J Pharmacol Exp Ther 1991; 256:432–440Medline, Google Scholar
113 Mody I, Heinneman U: NMDA receptors of dentate gyrus granule cells participate in synaptic transmission following kindling. Nature 1987; 326:701–704Crossref, Medline, Google Scholar
114 Mody I, Stanton PK, Heinneman U: Activation of N-methyl-d-aspartate receptors parallels changes in cellular and synaptic properties of dentate granule cells after kindling. J Neurophysiol 1988; 59:1033–1054Google Scholar
115 Sayin Ü, Rutecki P, Sutula T: NMDA-dependent currents in granule cells of the dentate gyrus contribute to induction but not permanence of kindling. J Neurophysiol 1999; 81:564–574Crossref, Medline, Google Scholar
116 Köhr G, De Koninck Y, Mody I: Properties of NMDA receptor channels in neurons acutely isolated from epileptic (kindled) rats. J Neurosci 1993; 13:3612–3627Google Scholar
117 Köhr G, Mody I: Kindling increases N-methyl-d-aspartate potency at single N-methyl-d-aspartate channels in dentate gyrus granule cells. Neuroscience 1994; 62:975–981Crossref, Medline, Google Scholar
118 Morrisett RA, Chow C, Nadler JV, et al: Biochemical evidence for enhanced sensitivity to N-methyl-d-aspartate in the hippocampal formation of kindled rats. Brain Res 1989; 496:25–28Crossref, Medline, Google Scholar
119 Martin D, Bowe MA, Nadler JV: A grease-gap method for studying the excitatory amino acid pharmacology of CA1 hippocampal pyramidal cells. J Neurosci Methods 1989; 29:107–114Crossref, Medline, Google Scholar
120 Martin D, McNamara JO, Nadler JV: Kindling enhances sensitivity of CA3 hippocampal pyramidal cells to NMDA. J Neurosci 1992; 12:1928–1935Google Scholar
121 Yeh G-C, Bonhaus DW, Nadler JV, et al: N-methyl-d-aspartate receptor plasticity in kindling: quantitative and qualitative alterations in the N-methyl-d-aspartate receptor-channel complex. Proc Natl Acad Sci USA 1989; 86:8157–8160Google Scholar
122 Yeh G-C: Ligand binding analyses of rat hippocampal N-methyl-d-aspartate receptor: allosteric interactions and receptor plasticity. Doctoral dissertation, Department of Pharmacology, Duke University, 1992Google Scholar
123 Nadler JV, Thompson MA, McNamara JO: Kindling reduces sensitivity of CA3 hippocampal pyramidal cells to competitive NMDA receptor antagonists. Neuropharmacology 1994; 33:147–153Crossref, Medline, Google Scholar
124 Lynch DR, Anegawa NJ, Verdoorn T, et al: N-methyl-d-aspartate receptors: different subunit requirements for binding of glutamate antagonists, glycine antagonists, and channel-blocking agents. Mol Pharmacol 1994; 45:540–545Medline, Google Scholar
125 Vezzani A, Speciale C, Della Vedova F, et al: Alternative splicing at the C-terminal but not at the N-terminal domain of the NMDA receptor NR1 is altered in the kindled hippocampus. Eur J Neurosci 1995; 7:2513–2517Google Scholar
126 Kraus JE, Nadler JV, McNamara JO: Regulation of alternative splicing of NMDAR1 in the kindling model. Mol Brain Res 1996; 41:97–104Crossref, Medline, Google Scholar
127 Kraus JE, McNamara JO: Measurement of NMDA receptor protein subunits in discrete hippocampal regions of kindled animals. Mol Brain Res 1998; 61:114–120Crossref, Medline, Google Scholar
128 Kraus JE: Molecular analyses of hippocampal NMDA receptors in the kindling model of epilepsy. Doctoral dissertation, Department of Neurobiology, Duke University, 1996Google Scholar
129 Tingley WG, Roche KW, Thompson AK, et al: Regulation of NMDA receptor phosphorylation by alternative splicing of the C-terminal domain. Nature 1993; 364:70–73Crossref, Medline, Google Scholar
130 Lau L-F, Huganir RL: Differential tyrosine phosphorylation of N-methyl-d-aspartate receptor subunits. J Biol Chem 1995; 34:20036–20041Google Scholar
131 Durand GM, Bennett MVL, Zukin RS: Splice variants of the N-methyl-d-aspartate receptor NR1 identify domains involved in regulation by polyamines and protein kinase C. Proc Natl Acad Sci USA 1993; 90:6731–6735Google Scholar
132 Kolaj M, Cerne R, Cheng G, et al: α subunit of calcium/calmodulin-dependent protein kinase enhances excitatory amino acid and synaptic responses of rat spinal dorsal horn neurons. J Neurophysiol 1994; 72:2525–2531Google Scholar
133 Lieberman DN, Mody I: Regulation of NMDA channel function by endogenous Ca2+-dependent phosphatase. Nature 1994; 369:235–239Crossref, Medline, Google Scholar
134 Wang L-Y, Orser BA, Brautigan DL, et al: Regulation of NMDA receptors in cultured hippocampal neurons by protein phosphatases 1 and 2A. Nature 1994; 369:230–232Crossref, Medline, Google Scholar
135 Wang YT, Salter MW: Regulation of NMDA receptors by tyrosine kinases and phosphatases. Nature 1994; 369:233–235Crossref, Medline, Google Scholar
136 Tong G, Shepherd D, Jahr CE: Synaptic desensitization of NMDA receptors by calcineurin. Science 1995; 267:1510–1512Google Scholar
137 Daigen A, Akiyama K, Otsuki S: Long-lasting change in the membrane-associated protein kinase C activity in the hippocampal kindled rat. Brain Res 1991; 545:131–136Crossref, Medline, Google Scholar
138 Kohira I, Akiyama K, Daigen A, et al: Enduring increase in membrane-associated protein kinase C activity in the hippocampal-kindled rat. Brain Res 1992; 593:82–88Crossref, Medline, Google Scholar
139 Moia LJMP, Matsui H, de Barros GAM, et al: Immunosuppressants and calcineurin inhibitors, cyclosporin A and FK506, reversibly inhibit epileptogenesis in amygdaloid kindled rat. Brain Res 1994; 648:337–341Crossref, Medline, Google Scholar
140 Westphal RS, Tavalin SJ, Lin JW, et al: Regulation of NMDA receptors by an associated phosphatase-kinase signaling complex. Science 1999; 285:93–96Crossref, Medline, Google Scholar
141 Kraus JE: Persistent NMDA receptor plasticity in the kindling model: a search for molecular mechanisms. Presented at Schizophrenia Conference: From Molecule to Public Policy, Santa Fe, NM, October 1998Google Scholar
142 Brown ME, Anton RF, Malcolm R, et al: Alcohol detoxification and withdrawal seizures: clinical support for a kindling hypothesis. Biol Psychiatry 1988; 23:507–514Crossref, Medline, Google Scholar
143 Booth BM, Blow FC: The kindling hypothesis: further evidence from a U.S. national study of alcoholic men. Alcohol Alcohol 1993; 28:593–598Medline, Google Scholar
144 Becker HC, Hale RL: Repeated episodes of ethanol withdrawal potentiate the severity of subsequent withdrawal seizures: an animal model of alcohol withdrawal “kindling.” Alcohol Clin Exp Res 1993; 17:94–98Crossref, Medline, Google Scholar
145 Ulrichsen J, Haugbol S, Brandt CF, et al: Irreversibility of kindled alcohol-withdrawal behavior in rats. Alcohol Alcohol 1998; 33:230–243Crossref, Medline, Google Scholar
146 Hu XJ, Ticku MK: Functional characterization of a kindling-like model of ethanol withdrawal in cortical cultured neurons after chronic intermittent ethanol exposure. Brain Res 1997; 767:228–234Crossref, Medline, Google Scholar
147 Becker HC, Veatch LM, Diaz-Granados JL: Repeated ethanol withdrawal experience selectively alters sensitivity to different chemoconvulsant drugs in mice. Psychopharmacol (Berl) 1998; 139:145–153Crossref, Medline, Google Scholar
148 Krugers HJ, Koolhaas JM, Bohus B, et al: A single social stress-experience alters glutamate receptor-binding in rat hippocampal CA3 area. Neurosci Lett 1993; 154:73–77Crossref, Medline, Google Scholar
149 Krystal JH, Karper LP, Seibyl JP, et al: Subanesthetic effects of the noncompetitive NMDA antagonist, ketamine, in humans. Arch Gen Psychiatry 1994; 51:199–214Crossref, Medline, Google Scholar
150 Malhotra AK, Pinals DA, Weingartner H, et al: NMDA receptor function and human cognition: the effects of ketamine in healthy volunteers. Neuropsychopharmacology 1996; 14:301–307Crossref, Medline, Google Scholar
151 Adler CM, Goldberg TE, Malhotra AK, et al: Effects of ketamine on thought disorder, working memory, and semantic memory in healthy volunteers. Biol Psychiatry 1998; 43:811–816Crossref, Medline, Google Scholar
152 Newcomer JW, Farber NB, Jevtovic-Todorovic V, et al: Ketamine-induced NMDA receptor hypofunction as a model of memory impairment and psychosis. Neuropsychopharmacology 1999; 20:106–118Crossref, Medline, Google Scholar
153 Lahti A, Koffel B, LaPorte D, et al: Subanesthetic doses of ketamine stimulate psychosis in schizophrenia. Neuropsychopharmacology 1995; 13:9–19Crossref, Medline, Google Scholar
154 Malhotra AK, Pinals DA, Adler CM, et al: Ketamine-induced exacerbation of psychotic symptoms and cognitive impairment in neuroleptic-free schizophrenics. Neuropsychopharmacology 1997; 17:141–150Crossref, Medline, Google Scholar
155 Mohn AR, Gainetdinov RR, Caron MG, et al: Mice with reduced NMDA receptor expression display behaviors related to schizophrenia. Cell 1999; 98:427–436Crossref, Medline, Google Scholar
156 Heresco-Levy U, Silipo G, Javitt DC: Gycinergic augmentation of NMDA receptor–mediated neurotransmission in the treatment of schizophrenia. Psychopharmacol Bull 1996; 32:731–740Medline, Google Scholar
157 Tsai G, Yang P, Chung L-C, et al: d-Serine added to antipsychotics for the treatment of schizophrenia. Biol Psychiatry 1998; 44:1081–1089Google Scholar
158 Goff DC, Tsai G, Levitt J, et al: A placebo-controlled trial of d-cycloserine added to conventional neuroleptics in patients with schizophrenia. Arch Gen Psychiatry 1999; 56:21–27Crossref, Medline, Google Scholar
159 Heresco-Levy U, Javitt DC, Ermilov M, et al: Efficacy of high-dose glycine in the treatment of enduring negative symptoms of schizophrenia. Arch Gen Psychiatry 1999; 56:29–36Crossref, Medline, Google Scholar
160 Farber NB, Newcomer JW, Olney JW: Glycine agonists: what can they teach us about schizophrenia? Arch Gen Psychiatry 1999; 56:13–17Google Scholar
161 Isokawa M, Avanzini G, Finch DM, et al: Physiologic properties of human dentate granule cells in slices prepared from epileptic patients. Epilepsy Res 1991; 9:242–250Crossref, Medline, Google Scholar
162 McDonald JW, Garofalo EA, Hood T, et al: Altered excitatory and inhibitory amino acid receptor binding in hippocampus of patients with temporal lobe epilepsy. Ann Neurol 1991; 29:529–541Crossref, Medline, Google Scholar
163 McNamara JO: Drugs effective in the therapy of the epilepsies, in Goodman and Gilman's The Pharmacological Basis of Therapeutics, 9th edition, edited by Hardman JG, Limbird LE, Molinoff PB, et al. New York, McGraw-Hill, 1996, pp 461–486Google Scholar
164 Rho JM, Donevan SD, Rogawski MA: Mechanism of action of the anticonvulsant felbamate: opposing effects on N-methyl-d-aspartate and gamma-aminobutyric acidA receptors. Ann Neurol 1994; 35:229–234Crossref, Medline, Google Scholar
165 Subramaniam S, Rho JM, Penix L, et al: Felbamate block of the N-methyl-d-aspartate receptor. J Pharmacol Exp Ther 1995; 273:878–886Medline, Google Scholar
166 White HS, Harmsworth WL, Sofia RD, et al: Felbamate modulates the strychnine-insensitive glycine receptor. Epilepsy Res 1995; 20:41–48Crossref, Medline, Google Scholar
167 Sutula TP: Reactive changes in epilepsy: cell death and axon sprouting induced by kindling. Epilepsy Res 1991; 10:62–70Crossref, Medline, Google Scholar
168 Kiessling M, Gass P: Immediate early gene expression in experimental epilepsy. Brain Pathol 1993; 3:381–393Crossref, Medline, Google Scholar
169 McNamara JO: Analyses of the molecular basis of kindling development. Psychiatry Clin Neurosci 1995; 49:S175–S178Google Scholar
170 Pollard H, Bugra K, Khrestchatisky M, et al: Seizure-induced molecular changes, sprouting and synaptogenesis of hippocampal mossy fibers. Epilepsy Res Suppl 1996; 12:355–363Medline, Google Scholar
171 Post RM, Weiss SRB: Kindling and stress sensitization, in Bipolar Disorder: Biological Models and Their Clinical Application, edited by Young LT, Joffe RT. New York, Marcel Dekker, 1997, pp 93–126Google Scholar
172 Mody I: Synaptic plasticity in kindling. Adv Neurol 1999; 79:631–643Medline, Google Scholar
173 Watanabe Y, Johnson RS, Butler LS, et al: Null mutation of c-fos impairs structural and functional plasticities in the kindling model of epilepsy. J Neurosci 1996; 16:3827–3836Google Scholar
174 Kojima N, Ishibashi H, Obata K, et al: Higher seizure susceptibility and enhanced tyrosine phosphorylation of N-methyl-d-aspartate receptor subunit 2B in fyn transgenic mice. Learning and Memory 1998; 5:429–445Medline, Google Scholar
175 Sprengel R, Suchanek B, Amico C, et al: Importance of the intracellular domain of NR2 subunits for NMDA receptor function in vivo. Cell 1998; 92:279–289Crossref, Medline, Google Scholar
176 Zheng D, Butler LS, McNamara JO: Kindling and associated mossy fibre sprouting are not affected in mice deficient of NGFI-A/NGFI-B genes. Neuroscience 1998; 83:251–258Crossref, Medline, Google Scholar
177 Bengzon J, Okabe S, Lindvall O, et al: Suppression of epileptogenesis by modification of N-methyl-d-aspartate receptor subunit composition. Eur J Neurosci 1999; 11:916–922Crossref, Medline, Google Scholar