Traumatic Axonal Injury: Novel Insights Into Evolution and Identification
Traumatic Brain Injury (TBI) is an often devastating and common problem that affects today's society. Only rough estimates of incidence and sequelae can be obtained, as many of the milder injuries go initially unreported. Current estimates note an incidence of 1.5 to 2 million a year in the United States, most commonly from motor vehicle accidents.1 It occurs bimodally in age groups from the teens through the twenties and after age 70. 80% are mild injury; 10%–13% moderate; and 7%–10% severe.2 A large portion of these surviving patients will have cognitive or emotional sequelae and will need neuropsychiatric interventions years after the injury. For example, 10%–75% of these patients have been reported to have depression.3 It is therefore essential to try to understand the pathophysiology of brain injury, be able to perform adequate diagnostic evaluations, and intervene as early as possible, in hopes of limiting continual injury processes.
In the last 20 years, improved clinical management has significantly reduced mortality, and for those who survive, new research has identified secondary injury processes that continue in the first hours and days postinjury that may be amenable to therapeutic intervention. Much of the current work in TBI focuses these processes and their therapeutic management potential. An excellent review of the history of research in acute brain injury was recently written.4 Beginning with a review of the anatomy of the neuron, its subcellular structure, and normal function, the authors discuss the biomechanics of the forces of TBI (rotational and translational), the implications of injury impact location, and the microscopic sequalae of the initial impact. Primary, secondary, and tertiary processes of injury to axons are emphasized. Difficulties in creating laboratory models of acceleration/deceleration injury, the problems associated with current research strategies, and how the theories of axonal injury have changed over the years are also addressed. The review closes with a consideration of imaging techniques for the acute TBI patient as well as potential interventions that may be on the horizon.4 This current communication focuses on three new concepts—new information regarding microscopic pathophysiology of secondary axonal injury; new diagnostic tests to quantify injury; and new imaging techniques that better identify axonal injury in TBI patients.
DIFFUSE AXONAL INJURY—MICROSCOPIC EVALUATION
Diffuse Axonal Injury (DAI) is a term applied to TBI-induced scattered destruction of white matter tracts. It was first described in 1955 by Lindenberg in patients with post traumatic hemorrhage of the corpus callosum.5 DAI can only be definitively diagnosed postmortem. DAI, as detected via histological assessment, is generally associated with shearing injuries especially those involving acceleration/deceleration. Typically, these patients show significant morbidity without evidence of mass lesions on early imaging. Correlation between axonal injury and the presence of neuropsychological abnormalities, even years after injury, have been described [for review see6].
Recent research has determined that initial axonal injury is an ongoing process, evolving over several hours to days. When first identified almost 50 years ago in postmortem analysis using silver salts, the finding of grossly swollen axonal bulbs in humans suggested that the axons were mechanically torn at the moment of injury, with an expulsion of their axoplasm to form the axonal bulb.4 Experimental studies in animals as well as humans, by Povlishock and colleagues in the early eighties, rejected this premise, showing that the forces of injury focally perturbed the axon, leading to impaired axonal transport that then led to focal axonal swelling, followed over several hours, by axonal disconnection.7 These findings have been confirmed by multiple laboratories leading to universal acceptance of the phenomenon that diffuse axonal injury involves progressive/evolving change and ultimately leads to axonal disconnection many hours postinjury.
In addition to the recognition of the evolving changes associated with DAI, Povlishock and colleagues have also followed the downstream consequences of the axonal disconnection showing that the diffuse axonal injury translates into diffuse synaptic terminal loss that then sets the stage for either adaptive or maladaptive neuroplasticity, perhaps explaining some of the morbidity found in these patients.7 Recognizing the evolving nature of diffuse axonal injury and its linkage to focally impaired axonal transport, more contemporary studies seeking to identify DAI in both the laboratory and forensic setting have relied on antibodies targeting amyloid precursor protein (APP), which is known to be delivered by axonal transport. Its anterograde passage is impeded by axonal injury, with subsequent accumulation in the axonal swelling. At present, antibodies to APP are the gold standard in both the experimental and forensic setting as well as in the evaluation of many preclinical drug studies focusing on the ability of various drugs to attenuate APP-positive swellings.4
While all of the research and clinical community have agreed that axonal swellings and disconnection are the consequence of injury and, as such, contribute to the adverse consequences of traumatic injury, new information arising from the experimental setting suggests that not all traumatically injured axons may go on to swell.8 This suggests that studies focusing on APP-positive swellings may underestimate the total number of axons injured by the traumatic event. This potential was first recognized by Povlishock and colleagues in studies seeking to better understand the subcellular mechanisms initiating traumatically induced axonal injury and swelling. Previous studies had shown that the traumatic episode perturbed the interaxonal cytoskeleton and impaired axonal transport. The assumption was advanced that these events were casually interrelated within the same axon. Recently, however, more critical assessment of these issues using double label immunofluorescent approaches, suggested that this premise was overly simplistic, underestimating the complexity of the pathobiology of DAI.9 Specifically, via double label approaches, some axons were recognized to demonstrate cytoskeletal alteration and detachment, without the subsequent formation of an axonal swelling. In contrast in other axons, axonal swelling occurred, sometimes independent of overt cytoskeletal damage. These studies have been subsequently confirmed via other approaches, collectively illustrating the complexity of the pathobiology of traumatically induced axonal change and the danger of relying on one individual marker of axonal injury to estimate the degree and extent of the traumatically induced axonal change.9,10 Preliminary studies conducted by Stone and colleagues in humans suggest that these same events described in animals are also ongoing in man, thereby suggesting the need for caution in human postmortem and forensic analyses that rely exclusively on markers targeting axonal swelling as the sole endpoint.9
CLINICAL INTERVENTIONS
Serum Markers
As novel discoveries are occurring on the pathological level, newer laboratory and imaging techniques are also finding use in the acute TBI setting. Recently two serum and cerebrospinal fluid (CSF) markers of central nervous system (CNS) injury have been studied in Europe. S-100B is an acidic calcium binding protein found in glial and Schwann cells with high specificity for CNS white matter. Neuron specific enolase (NSE) is an isoenzyme located in neurons, neuroendocrine cells, smooth muscle fibers, and adipose tissue. Both markers have been tentatively linked to the severity of the TBI and outcome predictability, particularly with severe injuries. The evidence for S-100B is considerably stronger than for NSE. Most authors agree that initial levels should be measured within 6 hours of the injury, certainly within the first day. Levels fall rapidly over the next few days. Levels have been measured in both CSF and, most commonly, in serum. A recent study suggests that to be strictly relevant to TBI, levels in CSF must be measured, as serum levels are affected by nonnervous system sources.11 In addition, another study has shown that serum levels of S-100B peak 2 days after CSF levels.12 As with most new research areas in medicine, the initial work has been with small groups of patients, study designs differ, studies have not controlled for the same preexisting factors, and outcome has generally been measured within 6 months of injury. Additionally, the primary outcome measure has been the Glasgow Outcome Scale (GOS), an 8-point scale that marks global states such as vegetative, dependency on others for activities of daily living, return to work, and minor deficits. It does not include in-depth neuropsychological or psychiatric testing.13
A recent comparison was done of CSF and serum S-100B and NSE levels to two immunological markers (sICAM-1 and Il-6; associated with CNS inflammatory response) and to clinical outcome and initial computed tomographic (CT) contusion size in 13 patients with Glasgow coma scale (GCS) scores of ≤ 8, as compared to 17 controls.14 Levels were measured over 14 days. Both CSF S-100B and NSE strongly correlated with contusion size. With the serum markers, only S-100B correlated with clinical outcome and contusion size in all 13 patients. Serum NSE correlated with the release of the immune factors only. The authors surmised that NSE was related to immune function and that S-100B can be a marker for severity of injury. They also noted that it is unclear if S-100B is protective or detrimental—as it may stimulate repair processes such as glial growth but also has been linked to deleterious calcium uptake. They did not control for previous psychiatric or neurological histories or alcohol use. A later study examined a larger cohort, 51 patients, with GCS < 9.15 Results again indicated that S-100B levels correlated with outcome, as measured by the GOS and by a quality of life self-assessment questionnaire. The authors measured outcome over a wide time range (5–24 months) and did not control for previous psychiatric or alcohol histories. However, both these studies confirmed the results of several groups from the 1990's that proposed the predictive value of S-100B in severe brain injury.
The predictive value of these markers is more controversial in mild brain injury. Serum S-100B and NSE were sampled in 66 patients with both mild (GCS 13–15) and moderate-severe (GCS ≤ 12) injury and compared to both volumetric CT lesions and initial GCS score.16 Results indicated that, as in previous studies, the patients with moderate-severe injury had higher NSE and S-100B levels and had longer release of these agents than did those with mild injuries. Both groups, however, had initially higher levels than controls. The levels were measured serially over 3 days, and all levels correlated with volume of contusions on CT. Patients with previous CNS disorders were excluded. Previous history of alcohol abuse was not mentioned. The same group followed with a comparison of S-100B and NSE values in 69 patients with a median GCS of 13.17 Twenty-nine of the subjects completed neuropsychological testing at 2 weeks and 6 months. Both markers correlated with initial GCS scores and neither correlated with CT findings. Patients with both subacute and chronic neuropsychological deficits had higher NSE and S-100B values and longer release times of both markers. However, at both 2 weeks and 6 months only the S-100B correlations reached statistical significance. The authors did control for past neurological, psychiatric, alcohol, and drug abuse histories. The conclusions were that, although both markers were elevated, S-100B was a more reliable marker of injury in mild TBI.
Two other groups have found correlations with outcome in larger cohorts of mildly injured patients (GCS 13–15). Townend found inverse correlation between initial S-100B levels and GOS scores at 1 month postinjury in 119 patients completing the study.13 deKruijk et al. examined S-100B and NSE levels in 104 mild TBI patients as compared to 91 controls.18 Median NSE levels in the patients were only slightly higher while S-100B levels were significantly higher than controls. Initial vomiting was also associated with higher S-100B levels. A self-assessment questionnaire of posttraumatic complaints was obtained at follow up in 79 of the 104 subjects.19 Within this group, a twofold increase in severity of cognitive and vegetative complaints was associated with higher initial serum levels of S-100B and NSE respectively. Savola et al.20 obtained serum S-100B levels within 6 hours of injury in 172 cases of mild TBI (GCS 13–15) and found that elevated levels (>0.5 μg/L) correlated with postconcussive symptoms reported at 2–6 weeks post injury. Only 50% of the 8 patients with CT-confirmed intracranial lesions had elevated S-100B levels.
Other areas of study with S-100B included an initial comparison to magnetic resonance (MR) imaging findings and development of appropriate cut-off values for normal and pathological levels of this marker. In 1999, Ingebrigtsen and colleagues found that the proportion with higher serum S-100B levels was increased in patients with MRI findings of contusion and observed a trend towards positive findings on neuropsychological testing at 3 months.21 However, only 14 of the 50 cohorts had detectable levels of S-100B and only 5 patients had MRI findings. Biberthaler compared 52 patients with mild injury (GCS 13–15) to 20 healthy controls and to 10 severely injured patients (GCS < 8).22 Results indicated that if 0.1ng/ml of serum S-100B was used as a cutoff for normal, then the test sensitively would reach 100% with 40% specificity for intracranial injury.
IMAGING STUDIES
Although it has been known for some time that MR imaging is more sensitive than CT in detecting axonal injury, both methods are widely used.5,6 CT examination, still the standard for life-threatening acute hemorrhage, is robust and relatively inexpensive. In contrast, while MR is much better for detection of white matter lesions, the exams take longer, are more expensive, and require special nonmagnetic ventilators, cardiac monitors, and other medical equipment. Both conventional and developing MR techniques can add value to the clinical assessment of TBI. Gradient echo (GE) images are sensitive to magnetic susceptibility (T2*) and can demonstrate even very small areas of hemorrhage.23–27 Such lesions are more easily visualized with this technique because the hemorrhagic blood creates a local magnetic field disturbance, causing a loss of signal. The number of small hemorrhagic lesions identified on T2* weighted GE images correlated with GCS score in several studies.23,26,27 In addition, lesion locations were appropriate to explain all focal neurological signs and symptoms in the acute phase (≤ 3 weeks) in one study.26 Nonhemorrhagic lesions are better visualized on T2 weighted spin echo sequences, especially those obtained using fluid attenuated inversion recovery (FLAIR), a technique that incorporates suppression of the bright signal from CSF. In a prospective study, 33 patients with normal CT scan but abnormal neurologic status underwent MR examination within 48 hours of injury.5 This group obtained T1 weighted echo planar, T2* weighted GE, T2 weighted FLAIR, and T2 weighted turbo spin echo images, and found that MR demonstrated more nonhemorrhagic lesions than CT. The authors noted that presence of nonhemorrhagic lesions was associated with a relatively good clinical outcome. However, outcome was measured only with the GOS, a relatively insensitive measure. It does not, for instance, include in-depth neuropsychological or neuropsychiatric evaluation. In another prospective study, a group of 21 patients with DAI underwent MR imaging within 24 hours of injury, then again on days 1, 3, 7, and 14.28 The signal intensity of the corpus callosum was measured on FLAIR images at each time point. The study found a positive correlation between the duration of unconsciousness and the maximum signal intensity of the corpus callosum, which occurred most commonly on day 7 (range 3–14). In addition, higher signal intensity was associated with an unfavorable outcome at 6 months, as determined by the GOS.
Newer types of MR imaging provide quantitative measures and show promise for increasing identification of DAI. A recent retrospective study examined 25 patients within 48 hours of TBI, comparing lesion visualization on T2 weighted fast spin echo, FLAIR, T2* weighted GE, and diffusion weighted (DW) images.25 Of 427 lesions identified on at least one type of image, 58% were detected on T2/FLAIR, 47% were detected on GE, and 22% were detected on both. DW imaging was the most sensitive, allowing visualization of 72%, including all nonhemorrhagic lesions. Importantly, 16% of lesions were visible only on DW imaging. All of these were hyperintense in appearance. The apparent diffusion constant (ADC) was decreased in the majority of lesions visible on DWI, indicating more restricted diffusion, perhaps an indication of cytotoxic edema. Approximately one-quarter displayed increased ADC, which may indicate the development of vasogenic edema. Consistent with expectation, many hemorrhagic lesions (23%) were seen only on the GE images. No follow up data was presented, so the clinical significance of the lesions identified could not be assessed.
Three studies used diffusion tensor imaging (DTI) to evaluate DAI. One measured diffusion anisotropy of the corpus callosum (splenium, body, genu) in 10 patients 2 weeks to 8 months after TBI (initial GCS scores ranged from 3 to 14) compared to normal individuals.29 Diffusion anisotropy was decreased by an average of approximately 25% in at least one region of the corpus callosum in all 10 patients, indicating disruption of the normally highly ordered structure. Decreased anisotropy was found even in areas that looked normal on both FLAIR and GE images. No correlation with functional measures was provided. A case report of a child with hemiparesis subsequent to a severe TBI (comatose for 3 days) reported that abnormalities on conventional MR did not correlate with clinical symptoms.30 DTI demonstrated decreased anisotropy in the cerebral peduncle of the midbrain. A preliminary study evaluated diffusion anisotropy of areas that appeared normal on standard CT and MR imaging in 5 patients with mild TBI (initial GCS score 13 to 15).31 All were imaged within the first 24 hours and 2 were imaged again at one month. There were areas of reduced anisotropy in regions that appeared normal by other imaging techniques in all 5 patients, most often in the internal capsule and corpus callosum (Figure 1). The authors noted that the decreased anisotropy in areas without edema or hemorrhage (which would have been seen on the other types of images gathered) indicate that diffusion parallel to the main axis of the axons has become impeded. They suggested misalignment of axonal membranes and of the cytoskeletal network as possible causes. By 30 days, some areas had returned to normal values, while others were still decreased. The authors suggested that these patterns may correspond to areas in which repair processes have been successful versus areas in which injury has progressed. All these studies indicate that various measures of water diffusion may be more sensitive to DAI than conventional MR imaging.
Magnetization transfer (MT) imaging is another quantitative MR technique that has been found to be related to tissue structure and thus can be used to assess structural integrity. In one study of DAI in an animal model, MT imaging results were found to be related to pathologically-proven injury.32 In a later study conducted by the same group, 28 patients who had experienced loss of consciousness as a result of head injury were compared to 15 controls.33 Most were first imaged within 2 weeks of injury (range 1 to 29 days). Follow up scans were obtained in 10 patients. Magnetization transfer ratios (MTRs) were calculated for all white matter areas that were abnormal on the T2 weighted images as well as for areas of normal appearing white matter. Outcome measures (GOS) were obtained 3 months to 3 years after injury. Decreased MTR was found in normal appearing white matter only in patients that had persistent functional deficits at follow up. However, absence of decrease in MTR in normal appearing white matter was found in patients with outcomes that ranged from very good to poor. In a subsequent related study, 13 patients who had experienced mild TBI were examined with conventional MR imaging and MT imaging several months to several years following injury.34 Neuropsychological testing was done in 9 patients, all of whom were found to be impaired. Most of the patients in the study (12/13) had negative conventional MR imaging but all were symptomatic. Contour mapping of the MTR revealed areas of profoundly decreased MTR in the splenium of the corpus callosum in 4 of these patients (Figure 2). A moderate correlation was found between a test of verbal learning and MTR for the splenium. Results of this study suggested that MT imaging may be sensitive to mild TBI when other modalities fail. In another study from this group, MT imaging and 1H (proton) MR spectroscopy of the splenium of the corpus callosum were obtained in 30 patients with TBI.35 Initial GCS scores ranged from 3 to 15 (mean score, 11). Assessment was done between 2 and 1129 days after injury. Outcome (GOS) was assessed a minimum of 3 months after injury. No correlation between MT imaging and outcome was found. However, a decreased N-acetylaspartate to creatine ratio (a possible indication of neuronal loss) was associated with poorer outcome. These results are consistent with prior suggestions that abnormal MR spectroscopy results may predict a poor clinical outcome.36 Limitations of this study include the wide range of times from injury to examination and injury to assessment of outcome.
CONCLUSION
In summary, new research techniques are beginning to make it clear that brain injury is not static but rather an evolving process. A better understanding of the nature of these events should spur the development of more effective interventions. Earlier, more sensitive methods of identifying and categorizing degrees of brain injury have the potential to better predict outcome, and thus guide clinical management.
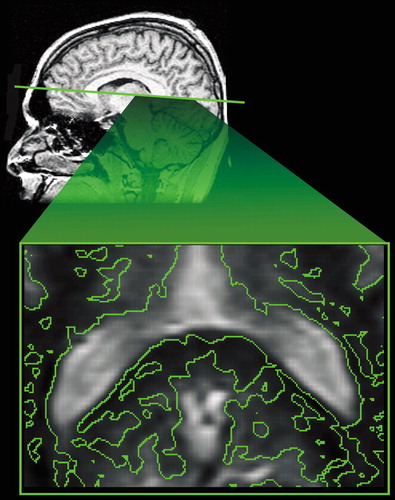
Cover. (Bottom) An axial contour map (irregular green lines) of areas that are more than 2 standard deviations below normal white matter magnetization transfer ratio in the region of the splenium of the corpus callosum in a patient with traumatic brain injury. (Top) The approximate axial section is indicated (straight green line) on a normal sagittal T1 weighted magnetic resonance (MR) scan.
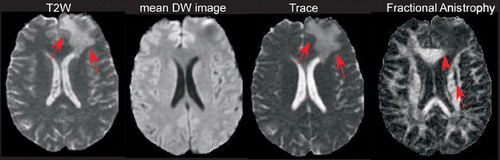
Figure 1. Axial Diffusion Tensor Imaging. An area with vasogenic edema is visible in the left frontal lobe on the T2 weighted image (arrows). It is much less evident on the mean diffusion-weighted map of the same section, produced by averaging the diffusion-weighted images obtained in all 23 noncolinear directions of the diffusion gradients. The corresponding trace map shows increased diffusivity in the left frontal lobe (arrows). In the fractional anisotropy map of the same section decreased anisotropy is visible in the left anterior portion of the corpus callosum due to the presence of vasogenic edema (arrowhead). Diffusion anisotropy is also decreased in the anterior portion of the left internal and external capsules (arrow), although these regions are characterized by normal T2 and trace values.
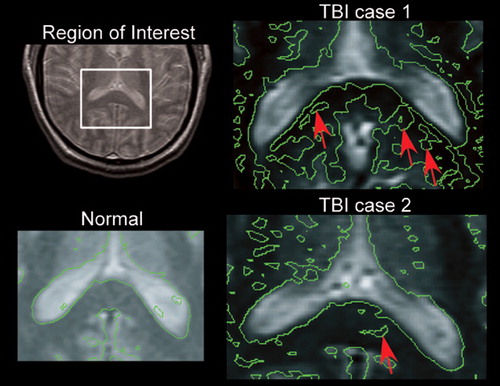
Figure 2. Axial Magnetization Transfer Imaging. A box indicates the region of the splenium of the corpus callosum in which a detailed analysis was performed by overlaying contours that correspond to 2 standard deviations (SD) below normal white matter magnetization transfer ratio (MTR). Arrows indicate regions in which the MTR was more than 2 SD below normal in these two traumatic brain injury (TBI) cases. Both patients had cognitive impairments consistent with TBI.
1 Consensus conference. Rehabilitation of persons with traumatic brain injury. JAMA 1999; 282:974–983Crossref, Medline, Google Scholar
2 Arciniegas DB, Held K, Wagner P: Cognitive impairment following traumatic brain injury. Curr Treat Options Neurol 2002; 4:43–57Crossref, Medline, Google Scholar
3 Hurley RA, Taber KH: Emotional disturbances following traumatic brain injury. Curr Treat Options Neurol 2002; 4:59–75Crossref, Medline, Google Scholar
4 Sahuquillo J, Poca MA: Diffuse axonal injury after head trauma. A review. Adv Tech Stand Neurosurg 2002; 27:23–86Crossref, Medline, Google Scholar
5 Paterakis K, Karantanas AH, et al: Outcome of patients with diffuse axonal injury: the significance and prognostic value of MRI in the acute phase. J Trauma 2000; 49:1071–1075Crossref, Medline, Google Scholar
6 McAllister TW, Sparling MB, et al: Neuroimaging findings in mild traumatic brain injury. J Clin Exp Neuropsychol 2001; 23:775–791Crossref, Medline, Google Scholar
7 Povlishock JT: Pathophysiology of neural injury: therapeutic opportunities and challenges. Clin Neurosurg 2000; 46:113–126Medline, Google Scholar
8 Stone JR, Walker SA, Povlishock JT: The visualization of a new class of traumatically injured axons through the use of a modified method of microwave antigen retrieval. Acta Neuropathol 1999; 97:335–345Crossref, Medline, Google Scholar
9 Stone JR, Singleton RH, Povlishock JT: Intra-axonal neurofilament compaction does not evoke local axonal swelling in all traumatically injured axons. Exp Neurol 2001; 172:320–331Crossref, Medline, Google Scholar
10 Hoshino S, Kobayashi S et al: Multiple immunostaining methods to detect traumatic axonal injury in the rat fluid-percussion brain injury model. Neurol Med Chir (Tokyo) 2003; 43:165–173Crossref, Medline, Google Scholar
11 Kleine TO, Benes L, Zofel P: Studies of the brain specificity of S100B and neuron-specific enolase (NSE) in blood serum of acute care patients. Brain Res Bull 2003; 61:265–279Crossref, Medline, Google Scholar
12 Petzold A, Keir G et al: Cerebrospinal fluid (CSF) and serum S100B: release and wash-out pattern. Brain Res Bull 2003; 61:281–285Crossref, Medline, Google Scholar
13 Townend WJ, Guy MJ et al: Head injury outcome prediction in the emergency department: a role for protein S-100B? J Neurol Neurosurg Psychiatry 2002; 73:542–546Crossref, Medline, Google Scholar
14 Pleines UE, Marganti-Kossmann MC et al: S-100B reflects the extent of injury and outcome, whereas neuronal specific enolase is a better indicator of neuroinflammation in patients with severe traumatic brain injury. J Neurotrauma 2001; 18:491–498Crossref, Medline, Google Scholar
15 Woertgen C, Rothoerl D, Brawanski A: Early S-100B serum level correlates to quality of life in patients after severe head injury. Brain Injury 2002; 16:807–816Crossref, Medline, Google Scholar
16 Herrmann M, Jost S et al: Temporal profile of release of neurobiochemical markers of brain damage after traumatic brain injury is associated with intracranial pathology as demonstrated in cranial computed tomography. J Neurotrauma 2000; 17:113–122Crossref, Medline, Google Scholar
17 Herrmann M, Curio N et al: Release of biochemical markers of damage to neuronal and glial brain tissue is associated with short and long term neuropsychological outcome after traumatic brain injury. J Neurol Neurosurg Psychiatry 2001; 70:95–100Crossref, Medline, Google Scholar
18 de Kruijk JR, Leffers P et al: S-100B and neuron-specific enolase in serum of mild traumatic brain injury patients. Acta Neurol Scand 2001; 103:175–179Crossref, Medline, Google Scholar
19 de Kruijk JR, Leffers P et al: Prediction of post-traumatic complaints after mild traumatic brain injury: early symptoms and biochemical markers. J Neurol Neurosurg Psychiatry 2002; 73:727–732Crossref, Medline, Google Scholar
20 Savola O, Hillborn M: Early predictors of post-concussion syndrome in patients with mild head injury. Eur J Neurol 2003; 10:175–181Crossref, Medline, Google Scholar
21 Ingebrigtsen T, Waterloo K et al: Traumatic brain damage in minor head injury: Relation of serum S-100 protein measurements to magnetic resonance imaging and neurobehavioral outcome. Neurosurgery 1999; 45:468–475Crossref, Medline, Google Scholar
22 Biberthaler P, Mussack T et al: Evaluation of S-100b as a specific marker for neuronal damage due to minor head trauma. World J Surg 2001; 25:93–97Crossref, Medline, Google Scholar
23 Tong KA, Ashwal S et al: Hemorrhagic shearing lesions in children and adolescents with posttraumatic diffuse axonal injury: improved detection and initial results. Radiology 2003; 227:332–339Crossref, Medline, Google Scholar
24 Kuzma BB, Goodman JM: Improved identification of axonal shear injuries with gradient echo MR technique. Surg Neurol 2000; 53:400–402Medline, Google Scholar
25 Huisman TAGM, Sorensen AG et al: Diffusion-weighted imaging for the evaluation of diffuse axonal injury in closed head injury. J Comput Assist Tomogr 2003; 27:5–11Crossref, Medline, Google Scholar
26 Yanagawa Y, Tsushima Y et al: A quantitative analysis of head injury using T2*-weighted gradient-echo imaging. J Trauma 2000; 49:272–277Crossref, Medline, Google Scholar
27 Scheid R, Preul G et al: Diffuse axonal injury associated with chronic traumatic brain injury: Evidence from T2*-weighted gradient-echo imaging at 3 T. Am J Neuroradiol 2003; 24:1049–1056Medline, Google Scholar
28 Takaoka M, Tabuse H et al: Semiquantitative analysis of corpus callosum injury using magnetic resonance imaging indicates clinical severity in patients with diffuse axonal injury. J Neurol Neurosurg Psychiatry 2002; 73:289–293Crossref, Medline, Google Scholar
29 Chan JH, Tsui EY et al: Diffuse axonal injury: detection of changes in anisotropy of water diffusion by diffusion-weighted imaging. Neuroradiology 2003; 45:34–38Crossref, Medline, Google Scholar
30 Zi L, Byun WM et al: Diffusion tensor magnetic resonance imaging of microstructural abnormalities in children with brain injury. Am J Phys Med Rehabil 2003; 82:556–559Medline, Google Scholar
31 Arfanakis K, Haughton VM et al: Diffusion tensor MR imaging in diffuse axonal injury. Am J Neuroradiol 2002; 97:568–575Google Scholar
32 McGowan JC, McCormack TM et al: Diffuse axonal pathology detected with magnetization transfer imaging following brain injury in the pig. Magn Reson Med 1999; 41:727–733Crossref, Medline, Google Scholar
33 Bagley LJ, McGowan JC et al: Magnetization transfer imaging of traumatic brain injury. J Magn Reson Imaging 2000; 11:1–8Crossref, Medline, Google Scholar
34 McGowan JC, Yang JH et al: Magnetization transfer imaging in the detection of injury associated with mild head trauma. Am J Neuroradiol 2000; 21:875–880Medline, Google Scholar
35 Sinson G, Bagley LJ et al: Magnetization transfer imaging and proton MR spectroscopy in the evaluation of axonal injury: Correlation with clinical outcome after traumatic brain injury. Am J Neuroradiol 2001; 22:143–151Medline, Google Scholar
36 Brooks WM, Friedman SD, Gasparovic C: Magnetic resonance spectroscopy in traumatic brain injury. J Head Trauma Rehabil 2001; 16:149–164Crossref, Medline, Google Scholar