Volume Transmission in the Brain: Beyond the Synapse
Abstract
A better understanding of volume transmission is important in neuropsychiatry, as this is now believed to be a major mode of action for many neuroactive substances, including the modulatory neurotransmitters and many psychiatric medications.1,17–19 The original conceptualization of information transfer in the central nervous system (CNS) was that it depended entirely on synaptic transmission. Although the point-to-point (wired) communication provided by synaptic transmission still receives most of the attention, there is growing evidence of the importance of other mechanisms, particularly extrasynaptic volume transmission. Volume transmission functions quite differently from synaptic (wired) transmission, which is optimized for communication that is high-speed and precise. In contrast, volume transmission provides a communication mode that is temporally slower and anatomically broader in reach, and more suited to modulatory and tuning functions.
History
The concept of volume transmission as an important mode of chemical communication in the brain was introduced in the 1980s.1,3,17–20 New techniques (e.g., receptor autoradiography, immunohistochemistry) that allowed detailed mapping of the locations of neurotransmitters (NTs) and their receptors led to several experimental findings that were not compatible with a purely synaptic mode of operation. Mapping studies found relative mismatches in some brain areas between the density of nerve terminals positive for a particular NT and the density of receptors for that NT. Situations in which there were very few terminals, as compared with the number of receptors, indicated that active substances must be getting to receptors in some manner other than release from an adjacent terminal. Studies demonstrating presence of NTs outside of synapses under several sets of experimental conditions (e.g., release enhanced, reuptake blocked) indicated that spillover from the synaptic cleft into the surrounding extracellular space (ECS) was one possible source.2 After release, diffusion of NTs through the ECS is likely to be sufficient to activate nonsynaptic receptors for a considerable distance (Figure 1).1–3 Mapping studies have identified axon terminals without postsynaptic specializations. Also, varicosities containing granules filled with monoamine NTs have been localized, not only in axon terminals, but also in other portions of neurons (e.g., dendrites, soma, non-terminal axon segments). These findings support the possibility of nonsynaptic release of NTs into the ECS.1,3,20 Nonsynaptic release was also supported by the finding of measurable levels of specific NTs in the ECS in regions lacking synaptic terminals for that NT.20 On the basis of animal (primarily rodent) studies, it was concluded that the modulatory NTs (e.g., acetylcholine, norepinephrine, dopamine, serotonin) act in great part by volume transmission because the majority of their NT-containing varicosities are not part of a synapse, and many of their projections do not make synaptic contact.1 This finding is supported by a recent study in nonhuman primates, reporting that 94% of tyrosine hydroxylase-positive boutons in prefrontal cortex Area 10 had no identifiable synaptic structures, indicating primarily nonsynaptic release of dopamine.21 There may be species-specific differences, as one study found a much higher percentage of acetylcholine-containing varicosities associated with synapses in the human cerebral cortex (67%) than had been reported for the rat (15%).22 Mechanisms for release of NT from nonsynaptic varicosities include exocytosis (similar to synaptic release) and reverse operation of NT transporters.1,17–20 Many other neuroactive substances (e.g., glial transmitters, peptides, nitric oxide, neurosteroids) are also released into the ECS.1,17–19 There is growing recognition that volume transmission is an important mode of action for other NTs (e.g., glycine, glutamate, GABA), as well.23–26 The location of a receptor, compared with the source of the neuroactive substance, is likely to be very important in determining activation.1–3 Many receptors that are not part of a synapse are located sufficiently far from the synaptic cleft that they will be unable to detect individual release events. Rather, they will experience a summation of surrounding events. Thus, they are more likely to signal slower (tonic) changes that are occurring over a larger area.
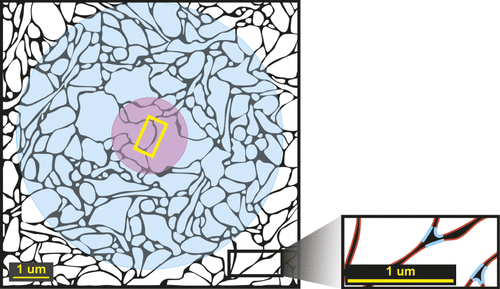
Local Volume Transmission
At the local level, volume transmission occurs via movement of neuroactive substances through the interstitial (extracellular) fluid that fills the ECS.4,27 On the microscopic scale, the physical makeup of the ECS will influence the speed and, in some cases, direction that substances and interstitial fluid move.4,27 The ECS, which occupies about 20% of the tissue volume, has a very complex, three-dimensional (3D) structure. This lengthens the actual path (measured as tortuosity) that a molecule must traverse as it moves around and between structures (e.g., neurons, glia, blood vessels).4,27 Recent 3D reconstructions (rat hippocampus) have allowed identification of two major structural patterns within the ECS differing in width, confirming an earlier conclusion by Van Harreveld (Figure 1).4,5 In areas where adjacent cell membranes are parallel, the ECS is narrower, constraining movement of interstitial fluid and molecules. Areas where several membranes converge are wider, allowing faster movement.5 Many factors can potentially affect the speed of movement of a neuroactive substance within the parenchyma of the brain, including its concentration and molecular size, binding to receptors or transporters, uptake processes, localized gradients in electrical potential, pressure changes (e.g., due to arterial pulses), temperature gradients (e.g., between an area of brain that is active and surrounding tissue), and bulk flow.4,17–19,27 Most signaling by volume transmission via the ECS is short-distance, particularly within gray matter. It has been suggested that the highly linear nature of white-matter tracts may provide a faster route for interstitial fluid movement than occurs in gray-matter areas.4,14
Psychiatric medications, which must cross the blood–brain barrier, will distribute throughout the brain parenchyma and therefore are accessing sites of action by volume transmission.1 Also, it has been proposed that the therapeutic efficacy of CNS drugs such as NT reuptake-inhibitors is mediated primarily by effects at nonsynaptic receptors.1 One reason is that the majority of NT transporters are located extrasynaptically. Another is the drug-induced increased NT concentration in the ECS. NT receptor subtypes that are synaptic generally have a lower affinity, and therefore require a higher NT concentration for activation, than the subtypes that are nonsynaptic. After synaptic release, diffusion quickly reduces the NT concentration in the synaptic cleft below the level required to activate synaptic receptors. However, it is still sufficiently high in the ECS to activate nonsynaptic receptors (Figure 1).1,2 Nonsynaptic receptor subtypes may also initiate very different changes from those of synaptic receptors and so may provide targets for development of new therapeutics. For example, the lower-affinity NMDA receptor subtype (GluN2A), which is primarily synaptic, has been linked to neuroprotective effects, whereas the high-affinity NMDA receptor subtype (GluN2B), which is primarily nonsynaptic, has been linked to glutamate-mediated excitotoxicity.28 It has been suggested that the failure of nonselective NMDA antagonists in clinical trials is due to the simultaneous activation of both pathways.28
Distance Volume Transmission
Longer-distance chemical signaling also occurs.14,16,18,19,29,30 It was demonstrated in the 1980s that a tracer introduced into the cerebrospinal fluid (CSF) quickly distributed into the brain parenchyma, outlining the microvasculature.31 Transport within the CSF potentially allows neuroactive substances rapid access to areas of the brain distant from their point of entry into the CSF. CSF is continuously produced, with a rate of formation in the human sufficient to fully replace its volume several times per day.29,30,32,33 CSF flow provides access to distant areas of the brain because it enters the perivascular spaces (PVS, Virchow-Robin space) that surround all penetrating arterioles and venules (intracerebral extrinsic microvasculature).6–9,34 The PVS is bounded on the inner side by the basal laminae of the penetrating vessels and on the outer side by the continuation of the external glial-limiting membrane (glia limitans) on the surface of the brain (Figure 2). The PVS may provide a mechanism for coordinated changes in activity across multiple areas.29,30,35 It has been proposed, for example, that distribution by this route of CSF containing neuroactive substances is important for onset and maintenance of specific behavioral or motivational states (e.g., fear, appetite, mood, circadian rhythms).29,30

In recent animal studies (rodent, canine, nonhuman primate), it has been shown that even rather large-molecular-weight substances are carried into the brain by the flow of subarachnoid CSF into the PVS surrounding penetrating arterioles.12–16,27,36 Arterial pulsations are an important driving force, and movement of substances via the PVS route was virtually abolished by piercing the dura, indicating that hydraulic integrity is essential for maintaining this flow.36–38 Larger-molecular-weight substances were retained within the PVS, likely because of the filtering function of the encompassing astrocytic endfeet (Figure 2). Small lipophilic tracers were also retained within the PVS and rapidly cleared.37 Water and lower-molecular-weight substances in the periarterial PVS followed the basement lamina of the arterioles to the capillary bed, passing freely into the ECS along this route.12,13 There is some controversy on the path taken thereafter. Clearance via the basement membranes in the walls of arteries to the cervical lymph nodes is one reported route.13,38,39 Alternatively, clearance via the perivenous PVS into the subarachnoid CSF has been reported.12,36,37 Subarachnoid CSF is removed by uptake via arachnoid villi into the venous sinuses and via the perineuronal spaces surrounding cranial nerves (e.g., olfactory, optic) to drain into the nasal and cervical lymphatics.29,30,32,36 Although clearly present in many species, a recent study has questioned the importance of the second route in primates.14 The flow of CSF into the brain via the PVS may also provide a new route for CNS medication administration. Recent animal studies (nonhuman primate, canine, rodent) have demonstrated that substances can be delivered into the brain parenchyma after lumbar intrathecal injection (Figure 3).14–16
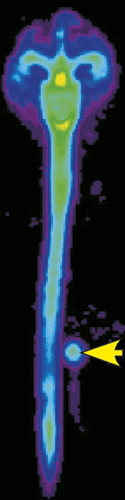

*Reproduced under the terms of the Creative Commons Attribution License.
Overall, this system provides a path by which substances can be both circulated throughout and removed from the brain parenchyma.12–16,30,36,38,39 A recent (rodent) animal study reported that the rate of flow was most rapid during sleep, slowing abruptly when animals were awakened.40 This was a result of a reduction in the volume fraction of the ECS, which decreased from about 22%−24% (asleep or anesthetized) to about 13%−15% (awake). As would be predicted, clearance of substances was much faster during sleep, when the ECS was largest. The authors noted that these results suggest that one important function of sleep is efficient clearance of toxins and wastes from the brain by this pathway.40 Any diminution in this flow through the ECS could impair clearance of solutes (e.g., amyloid beta), and this has been proposed as an important pathophysiological mechanism for several neurodegenerative diseases.12,38 It may also contribute to sequelae of low-level brain injury (microinfarction, mild traumatic brain injury).12,38 These conditions may trigger a prolonged widespread reactive gliosis and/or focal disruption of vascular perfusion. Both reactive gliosis and focal loss of vascular perfusion are associated with impaired bulk flow through the ECS, which may promote accumulation of neurotoxic substances.12,38 Volume transmission also provides a route for toxic substances in the blood or CSF to reach the brain parenchyma. This route has been implicated in both multiple sclerosis and viral encephalitis.30 It may also be the origin of the neuropsychiatric symptoms that can occur after some systemic infections.41
Conclusions
Recent scientific evidence is leading to a greater understanding of the complexities and intricacies of chemical communication in the brain. The importance and relevance of both fast/precise and slow/summative types of communication have yet to be fully appreciated. Advancing knowledge is gradually elucidating the roles that volume transmission plays in supporting normal function. Also, this mode of chemical communication provides new avenues with great potential for intervening in neurodegenerative conditions, after acute trauma, and in the development of novel therapeutics for chronic psychiatric conditions that, to date, have had limited available treatments.
1 : Non-synaptic receptors and transporters involved in brain functions and targets of drug treatment. Br J Pharmacol 2010; 160:785–809Crossref, Medline, Google Scholar
2 : Dopamine spillover after quantal release: rethinking dopamine transmission in the nigrostriatal pathway. Brain Res Brain Res Rev 2008; 58:303–313Crossref, Google Scholar
3 : Dopamine release in the basal ganglia. Neuroscience 2011; 198:112–137Crossref, Medline, Google Scholar
4 : Diffusion in brain extracellular space. Physiol Rev 2008; 88:1277–1340Crossref, Medline, Google Scholar
5 : Extracellular sheets and tunnels modulate glutamate diffusion in hippocampal neuropil. J Comp Neurol 2013; 521:448–464Crossref, Medline, Google Scholar
6 : Three-dimensional organization of the perivascular glial-limiting membrane and its relationship with the vasculature: a scanning electron microscope study. Okajimas Folia Anat Jpn 2010; 87:109–121Crossref, Medline, Google Scholar
7 : In-vivo 3D morphology of astrocyte–vasculature interactions in the somatosensory cortex: implications for neurovascular coupling. J Cereb Blood Flow Metab 2011; 31:795–806Crossref, Medline, Google Scholar
8 : Developmental aspects of the intracerebral microvasculature and perivascular spaces: insights into brain response to late-life diseases. J Neuropathol Exp Neurol 2011; 70:1060–1069Crossref, Medline, Google Scholar
9 : The human brain intracerebral microvascular system: development and structure. Front Neuroanat 2012; 6:38Crossref, Medline, Google Scholar
10 : Morphological characteristics and distribution pattern of the arterial vessels in human cerebral cortex: a scanning electron microscope study. Anat Rec 1998; 251:87–96Crossref, Medline, Google Scholar
11 : Morphological features in human cortical brain microvessels after head injury: a three-dimensional and immunocytochemical study. Anat Rec A Discov Cell. Evol Biol 2003; 273:583–593Google Scholar
12 : Is there a cerebral lymphatic system? Stroke 2013; 44(Suppl 1):S93–S95Crossref, Medline, Google Scholar
13 : Drainage of cells and soluble antigen from the CNS to regional lymph nodes. J Neuroimmune Pharmacol 2013; 8:840–856Crossref, Medline, Google Scholar
14 : Physiology of the intrathecal bolus: the leptomeningeal route for macromolecule and particle delivery to CNS. Mol Pharm 2013; 10:1522–1532Crossref, Medline, Google Scholar
15 : Evaluating glymphatic pathway function utilizing clinically relevant intrathecal infusion of CSF tracer. J Transl Med 2013; 11:107 [E-pub ahead of print]Crossref, Medline, Google Scholar
16 : CNS penetration of intrathecal-lumbar idursulfase in the monkey, dog, and mouse: implications for neurological outcomes of lysosomal storage disorder. PLoS ONE 2012; 7:e30341Crossref, Medline, Google Scholar
17 : Understanding wiring and volume transmission. Brain Res Brain Res Rev 2010; 64:137–159Crossref, Google Scholar
18 : The discovery of central monoamine neurons gave volume transmission to the wired brain. Prog Neurobiol 2010; 90:82–100Crossref, Medline, Google Scholar
19 : Extrasynaptic neurotransmission in the modulation of brain function: focus on the striatal neuronal–glial networks. Front Physiol 2012; 3:136Crossref, Medline, Google Scholar
20 : Extrasynaptic exocytosis and its mechanisms: a source of molecules mediating volume transmission in the nervous system. Front Physiol 2012; 3:319Crossref, Medline, Google Scholar
21 : The fine structure of the dopaminergic innervation of area 10 of macaque prefrontal cortex. Eur J Neurosci 2013; 37:1061–1071Crossref, Medline, Google Scholar
22 : Cholinergic synapses in human cerebral cortex: an ultrastructural study in serial sections. Exp Neurol 1997; 144:361–368Crossref, Medline, Google Scholar
23 : Visualization of glutamate as a volume transmitter. J Physiol 2011; 589:481–488Crossref, Medline, Google Scholar
24 : Mechanisms of glycine release, which build up synaptic and extrasynaptic glycine levels: the role of synaptic and non-synaptic glycine transporters. Brain Res Bull 2013; 93:110–119Crossref, Medline, Google Scholar
25 : The effects of volatile anesthetics on synaptic and extrasynaptic GABA-induced neurotransmission. Brain Res Bull 2013; 93:69–79Crossref, Medline, Google Scholar
26 : The role of non-synaptic extracellular glutamate. Brain Res Bull 2013; 93:17–26Crossref, Medline, Google Scholar
27 : Diffusion of macromolecules in the brain: implications for drug delivery. Mol Pharm 2013; 10:1492–1504Crossref, Medline, Google Scholar
28 : Role of nonsynaptic GluN2B-containing NMDA receptors in excitotoxicity: evidence that fluoxetine selectively inhibits these receptors and may have neuroprotective effects. Brain Res Bull 2013; 93:32–38Crossref, Medline, Google Scholar
29 : The regulation of brain states by neuroactive substances distributed via the cerebrospinal fluid; a review. Cerebrospinal Fluid Res 2010; 7:1Crossref, Medline, Google Scholar
30 : Volume transmission-mediated encephalopathies: a possible new concept? Arch Neurol 2012; 69:315–321Crossref, Medline, Google Scholar
31 : Evidence for a “paravascular” fluid circulation in the mammalian central nervous system, provided by the rapid distribution of tracer protein throughout the brain from the subarachnoid space. Brain Res 1985; 326:47–63Crossref, Medline, Google Scholar
32 : Anatomy and physiology of cerebrospinal fluid. Eur Ann Otorhinolaryngol Head Neck Dis 2011; 128:309–316Crossref, Medline, Google Scholar
33 : Brain superhighways. Sci Transl Med 2012; 4:147fs29Crossref, Medline, Google Scholar
34 : Meninges: from protective membrane to stem cell niche. Am J Stem Cells 2012; 1:92–105Medline, Google Scholar
35 : Volume transmission of beta-endorphin via the cerebrospinal fluid; a review. Fluids Barriers CNS 2012; 9:16Crossref, Medline, Google Scholar
36 : A paravascular pathway facilitates CSF flow through the brain parenchyma and the clearance of interstitial solutes, including amyloid B. Sci Transl Med 2012; 4:147ra111Crossref, Medline, Google Scholar
37 : Paravascular microcirculation facilitates rapid lipid transport and astrocyte signaling in the brain. Sci Rep 2013; 3:2582Crossref, Medline, Google Scholar
38 : Interstitial fluid drainage is impaired in ischemic stroke and Alzheimer’s disease mouse models. Acta Neuropathol 2013; 126:353–364Crossref, Medline, Google Scholar
39 : Afferent and efferent immunological pathways of the brain: anatomy, function, and failure. Brain Behav Immun 2013; [E-pub ahead of print]Medline, Google Scholar
40 : Sleep drives metabolite clearance from the adult brain. Science 2013; 342:373–377Crossref, Medline, Google Scholar
41 : Sepsis-associated encephalopathy: review of the neuropsychiatric manifestations and cognitive outcome. J Neuropsychiatry Clin Neurosci 2011; 23:237–241Link, Google Scholar