rTMS/iTBS and Cognitive Rehabilitation for Deficits Associated With TBI and PTSD: A Theoretical Framework and Review
Abstract
Rehabilitation of cognitive and psychosocial deficits resulting from traumatic brain injury (TBI) continues to be an area of concern in health care. Commonly co-occurring psychiatric disorders, such as major depressive disorder and posttraumatic stress disorder, create additional hurdles when attempting to remediate cognitive sequelae. There is increased need for procedures that will yield consistent gains indicative of recovery of function. Intermittent theta-burst stimulation (iTBS), a form of repetitive transcranial magnetic stimulation, has potential as an instrument that can be tailored to aid cognitive processes and support functional gains. The use of iTBS enables direct stimulation of desired neural systems. iTBS, performed in conjunction with behavioral interventions (e.g., cognitive rehabilitation, psychotherapy), may result in additive success in facilitating cognitive restoration and adaptation. The purpose of this theoretical review is to illustrate how the technical and physiological aspects of iTBS may enhance other forms of neurorehabilitation for individuals with TBI. Future research on combinatorial iTBS interventions has the potential to translate to other complex neuropsychiatric conditions.
Traumatic brain injuries (TBIs) can result in alterations in neurocognitive performance that negatively influence ability to complete instrumental activities of daily living (IADLs). Worldwide, 69 million people are estimated to sustain a TBI each year (1). Although 70%–90% of TBIs are mild (i.e., mTBI; concussions) with the cognitive consequences of injury typically resolving within 90 days (2, 3), a significant number of individuals with injuries across all levels of TBI severity continue to have persisting deficits that can reduce quality of life (1, 4, 5). Major depressive disorder (MDD) and posttraumatic stress disorder (PTSD) are two psychiatric disorders that commonly co-occur with TBI (6, 7). It is estimated that up to 53% of patients hospitalized after TBI also have MDD (6), and that 16.5% of individuals sustaining a TBI also have co-occurring PTSD (8). Impaired cognition is a frequently observed sequela of both MDD (9) and PTSD (10, 11). Coupled with a TBI, these psychiatric presentations may further exacerbate alterations to cognitive functioning. Rehabilitative programs and strategies intended to remediate cognitive processes such as executive-attentional functions, memory, and language show promise, but reported gains frequently fall short of restoring an individual’s baseline level of functional performance (12–16). As a result, many individuals continue to remain dependent on supportive devices, strategies, and accommodations when performing IADLs.
Transcranial magnetic stimulation (TMS) is a noninvasive method of neuromodulation. It has received Food and Drug Administration (FDA) approval for treating a number of disorders, including depression (17), obsessive-compulsive disorder (OCD) (18), and smoking addiction (19), and shows promise as a means of treating the cognitive and psychosocial effects of TBI (20–23). The purpose of this theoretical review is to provide evidence for the empirical basis for pairing TMS with behaviorally based interventions, such as cognitive rehabilitation (CR) exercises. The potential clinical benefits of this pairing will be discussed as they pertain to the rehabilitation of individuals with closed-head TBI, with and without co-occurring MDD and/or PTSD. The empirical framework detailed in this review consists of four key elements: identifying the population, establishing a potentiated neural environment using TMS and modulating neural responses, pairing TMS neurostimulation with behaviorally and cognitively based interventions (e.g., CR, psychotherapy) that target processes supported by the engaged neural targets, and enhancing transfer of treatment-related gains to performance of IADLs. In explicating this framework, the basic principles of TMS neuromodulation, specifically repetitive TMS (rTMS) and intermittent theta-burst stimulation (iTBS, a specific type of rTMS), and CR are examined with the goal of advancing the field of neurorehabilitation (Figure 1). For individuals with TBI and co-occurring mental health conditions, this review illustrates how the technical and physiological aspects of iTBS can be leveraged to enhance therapeutic effects of neurorehabilitation.
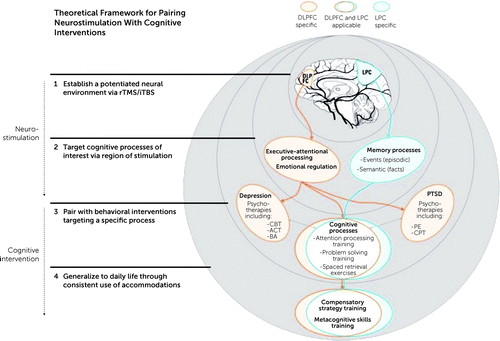
FIGURE 1. The model depicts a theoretical framework for the paired use of rTMS/iTBS with behavioral interventions targeting cognitive and psychosocial rehabilitationa
aCognitive interventions should be performed directly after stimulation to take advantage of the potentiated neural environment. Neural networks likely to be influenced by stimulation of the dorsolateral prefrontal cortex (DLPFC) include the default mode network, dorsal anterior network, salience network, and central executive network, also referred to as frontal central parietal network. Stimulation of the lateral parietal cortex (LPC) is likely to influence the hippocampal network. The model includes several examples of psychotherapies with strong empirical support, including, but not limited to cognitive-behavioral therapy (CBT), acceptance and commitment therapy (ACT), behavioral activation (BA), prolonged exposure (PE), and cognitive processing therapy (CPT). This list is not all-encompassing; it merely provides several common examples. rTMS, repetitive transcranial magnetic stimulation; iTBS, intermittent theta-burst stimulation. The brain image depicted is adapted from “Human Anatomy,” by BioRender.com (2020). Retrieved from https://app.biorender.com/biorender-templates.
Element 1: Population of Interest
Closed-head TBIs (nonpenetrating head injuries) can produce widespread neural damage; even those that are deemed mild may trigger metabolic dysfunction that alters neural processing (24–26) (Figure 2). Structural damage to neural networks is common, with TBI pathology capable of disrupting basic underlying cognitive function (25, 26). Behaviorally, deficits commonly manifest in the form of hampered executive-attentional functions and impaired memory (27, 28).
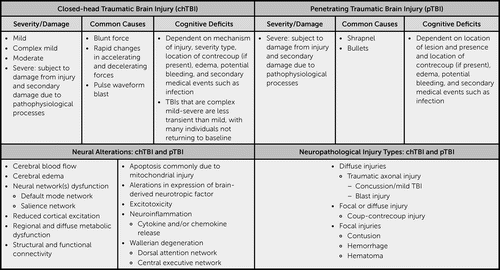
FIGURE 2. A traumatic brain injury synopsis
In many instances, TBIs are the onset of a cascade of medical events and are commonly accompanied by co-occurring MDD as well as PTSD. MDD symptoms may result from a combination of functional changes, the psychological toll that results from the TBI, and the immediate aftermath of the injury, as well as other related changes (e.g., increased pain, sleep changes); PTSD may result from the event in which the TBI occurred. When addressing a TBI, one must be cognizant that structural, functional, and metabolic changes may have occurred (29–38). Mechanistically, there can be substantial overlap between MDD and TBI in terms of neural disruption (6, 7), with both linked with an inflammatory response within the brain that negatively influences neural pathways, neurotransmitter release, growth factors, and neurogenesis (39–42). Glutamate and GABA are two of the neurotransmitters directly altered after a TBI (43) linked with MDD (44). Additionally, there are also higher levels of CSF cytokines (an acute inflammatory response) following moderate to severe TBI, which can be predictive of posttraumatic depression (45). Relatedly, there is evidence that markers of neural inflammation may also be present with PTSD (46). Up to 50% of individuals with PTSD may also present with MDD, strongly linking these two disorders (47). Collectively, these findings provide a strong theoretical rationale for why the two co-occur at such high rates. rTMS has the potential to modulate neuroinflammation (35) and increase production of neurotransmitters such as glutamate (48) (Table 1).
Common neural alterations due to TBI | TMS-induced response | Areas of note |
---|---|---|
Altered cerebral blood flow (29–31) | Altered cerebral blood flow in healthy individuals and persons with depression | Prefrontal cortex, posterior cingulate, thalamus, hippocampus, and parahippocampus |
Cerebral edema (32) | Reduced cerebral edema (rodent models) | Cerebral hemispheres |
Disruption of neurotransmitter regulation (33) | Modulation of dopamine | Ipsilateral anterior cingulate cortex and orbitofrontal cortex |
Regional and diffuse metabolic dysfunction (34, 35) | Increased expression of brain-derived neurotrophic factor (rodent models) | Dopamine, glutamate, and brain-derived neurotrophic factor |
Reduced cortical excitation (36, 37) | Increased cortical excitation for individuals with TBI | Cortex |
Functional connectivity (38) | Altered functional connectivity reducing symptoms for individuals with TBI | Dorsolateral prefrontal cortex, default mode network, and subgenual anterior cingulate cortex |
Neuroinflammation (35) | Reduction in neuroinflammation through modulation of cytokine levels (rodent model) | Prefrontal cortex and spinal cord tissue homogenates |
TABLE 1. Neural alterations associated with traumatic brain injury (TBI)a
Element 2: Creating a Potentiated Neural Environment
Given the strong overlap between TBI, MDD, and PTSD, it is important to investigate how treatments that have shown varying levels of success alleviating symptoms of one disorder (in this case MDD) may influence remediation of the sequelae more commonly linked with the others. rTMS is a neuromodulatory treatment approved for treatment-resistant MDD (17) that is a strong candidate as a potential medical intervention for other neurological impairments characterized by aberrant physiological and chemical functioning that disrupts neurological homeostasis (e.g., cognitive impairments secondary to TBI). rTMS delivers repeated trains of relatively focal stimulation provided via external magnetic coils, which create an electrical field that induces a current within the brain that triggers inhibitory or excitatory neural responses (49–51). The area of concern or presenting deficit is likely to guide the course of treatment (e.g., inhibitory stimulation to reduce aversive behaviors). The stimulation provided will be either more rapid (i.e., suprathreshold) or slower (i.e., subthreshold) than what is typically occurring physiologically, thereby inducing faster or slower neuronal depolarization and ultimately suppressing or facilitating neuronal excitability. The induced neural responses may facilitate an environment that is more responsive to modification, potentially enabling recovery of functions diminished by disease or injury and reducing aversive behaviors. Therefore, the corresponding stimulation is delivered in a manner consistent with the presenting deficit (e.g., inhibitory stimulation to reduce aversive behaviors).
Site of Stimulation
rTMS can lead to shifts in a number of physiological processes that may benefit cognitive function. A major area of concern in using rTMS is ensuring that stimulation is occurring in a location likely to induce positive changes. It is still a relatively novel treatment, and efficacy studies are actively attempting to determine which sites of stimulation are appropriate for a given condition. The dorsolateral prefrontal cortex (DLPFC) is a common target because it is a gateway to many high-level aspects of cognitive functioning with top-down connections to multiple neural systems of interest (Figure 1). Stimulation of the DLPFC is associated with alterations in regional cerebral blood flow (CBF) in a number of regions, including the prefrontal cortex, posterior cingulate, thalamus, hippocampus, and parahippocampus (29–31). These regions are pertinent for high-level cognitive functions; increased CBF has been observed to coincide with improved cognition as evaluated by assessments of memory and executive-attentional functioning (52). However, it must be noted that shifts in CBF may not necessarily be associated with cognitive improvement. Additionally, rTMS may also influence neurotransmitter release. Cho and Strafella (33) observed that 10 Hz of rTMS delivered to the left DLPFC induced ipsilateral dopamine release, which is particularly important considering that dopamine can influence multiple aspects of cognition (e.g., attention and motivation) (33, 53). Higher functional connectivity between the left DLPFC and striatum is linked with increased responsiveness to treatment among individuals with MDD (54).
Even with improvements in stimulation, precise targeting of specific regions and networks is an area that remains under investigation. Sack et al. (55) examined four commonly deployed TMS placement methodologies using a size congruity effect paradigm with stimulation delivered to the right parietal sulcus. They observed that use of individual fMRI-guided TMS neuronavigation produced a stronger behavioral effect relative to individual MRI-guided TMS neuronavigation, TMS guided by group functional Talairach coordinates, and TMS placement determined by 10–20 EEG position P4. Recent work by Herrold et al. (56) suggests that using multimodal imaging consisting of structural, functional, and diffuse MRI coupled with TMS-induced electric fields has the potential to benefit the recovery of individuals with TBI who have comorbid conditions. To further enhance treatment for depression, Luber et al. (57) proposed that fMRI-guided neuronavigation needs to be paired with activation of specific networks based on regions of dysfunction. They proposed priming systems supporting goal pursuit, specifically the promotion (MDD) and prevention systems (generalized anxiety disorder [GAD]). They contended that doing so could reveal individual differences enabling direct targeting of locations in the left (MDD) and right PFC (GAD). Participation in fMRI tasks likely to be impaired as a result of TBI may support identification of regions most likely to benefit from stimulation, allowing further individualization and enhancement of treatment.
In clinical practice for treatment-resistant depression, rTMS is commonly delivered at 10 Hz with an intensity of 120% of motor threshold for 3,000 pulses provided over the course of 5 days per week for 4–6 weeks (17, 58). An examination of two commonly implemented FDA-approved protocols for treatment-resistant MDD revealed that an H1-coil paired with pharmacological therapy was superior to a figure-8 coil paired with pharmacological therapy (59). It is important to note that both protocols were safely tolerated and efficacious relative to pharmacotherapy alone (59). Developments in the field have continued, with new techniques increasing treatment efficiency. For efficacy and safety reasons, initial studies targeted isolated areas. As more information is uncovered, treatment may commence by targeting the DLPFC with standard treatment protocols. Treatment may then progress to targeting additional sites with treatment protocols tailored to match the need to engage or inhibit neural functions as determined by neuroimaging findings and individual behavioral profiles.
Theta-Burst Stimulation
TBS is a form of rTMS that consists of pulses delivered at a much faster rate than used in standard rTMS. TBS has two dominant subcategories based on pattern of stimulation (intermittent and continuous). During iTBS, patterned pulses (theta bursts) are delivered at a high frequency, with intermittent pauses occurring between pulse sets (60, 61) (e.g., 100-µs paired pulses separated by 100-ms interpulse intervals). Use of iTBS increases neural excitability (61, 62). Alternatively, in continuous TBS (cTBS), pulses are delivered without pauses, which commonly reduces excitability (62).
Collectively, the potentiated neural environment created by both rTMS and cTBS theoretically may be more adaptable to change and can produce enduring effects, in that high- and low-frequency stimulation are thought to induce long-term potentiation (LTP) or long-term depression (LTD) mechanisms. These are the neurobiological processes that enable persisting functional neuronal changes after cessation of stimulation (63–65). Specifically, LTP is an activity- or stimulus-driven increase in synaptic strength that is frequently discussed in relation to memory and learning, whereas LTD is a related phenomenon involving a reduction in synaptic strength (66, 67).
The particular use of theta waves in neurostimulation research is, in part, a product of hippocampal research. Entorhinal inputs producing theta waves have been shown to modulate neurons and synaptic weights influencing memory (68). iTBS can induce cortical potentiation lasting up to 60 minutes after treatment cessation (69). The prolonged period provides additional opportunities for the stimulated pyramidal neurons and interneurons to undergo depolarization and subsequently increase in synaptic strength to engage the LTP-like mechanisms that are important to memory and learning (62, 70). Induction of LTP may be particularly beneficial clinically because these mechanisms are commonly impaired after a TBI.
Although both traditional rTMS and iTBS are effective at inducing LTP and LTD, the iTBS pattern enables a shorter administration time (approximately 3–6 minutes as opposed to 30–38 minutes for traditional rTMS), allowing for more pulses to be delivered daily and reducing the number of treatment sessions needed (60, 71, 72). Findings from work comparing iTBS to traditional rTMS protocols with regard to treating MDD indicate that the two methods yield similar positive outcomes (60, 73), with iTBS notably more efficient and cost effective (71). With respect to safety, researchers have noted that iTBS produces similar side effects in comparison to traditional rTMS (e.g., nausea, fatigue, insomnia); the most common side effect reported is headache (73). Furthermore, a review of TMS use, including use with special populations, noted the risk of seizure related to TMS use was less than 1% (74).
Elements 3 and 4: Pairing rTMS/iTBS With Behaviorally and Cognitively Based Interventions
As noted, use of rTMS and iTBS creates a neural environment that is potentially malleable and primed for recovery, which may prove beneficial to processes impaired following neurological trauma. After cessation of iTBS to the DLPFC, enhanced neuroplasticity is thought to last for at least 60 minutes, during which time behaviorally and cognitively based inventions such as CR and psychotherapy can be provided. This window of time provides an opportunity to target specific cognitive processes with the goal of triggering engagement of activity-dependent neural mechanisms of synaptic change (i.e., metaplasticity). These mechanisms are important because they serve as adaptive neural processes and are likely to aid recovery of impaired cognitive functions, particularly when paired with behavioral interventions targeting specific areas of cognition.
Why Not Just Neuromodulation in Isolation?
Research directly examining the influence of rTMS and iTBS on the cognition of individuals with a history of TBI is limited, and findings are mixed. Treatment studies of individuals with a history of severe TBI who present with a disorder of consciousness reveal that use of rTMS is associated with varying levels of success, including neurobehavioral gains such as a positive shift in cognitive state (i.e., cognitive responses consistent with movement toward conscious engagement) (20, 21). Studies examining stimulation of the motor cortex report no substantial gains in cognitive function (75–77), underscoring the importance of the site of stimulation. Studies of less severe TBI populations have also produced mixed results. Both Lee and Kim (22) and Hoy and colleagues (23) observed improvement in areas of executive functioning, whereas research by Neville and colleagues (78) and Koski and colleagues (79) both observed minimal to no changes with respect to cognitive performance. It should be noted that Hoy and colleagues (23) did not find a significant benefit with respect to alleviating symptoms of depression relative to a placebo treatment, which was the primary aim of the study. The variability observed with respect to gains following rTMS may be related to the precision of neural targeting with stimulation of the target site pertinent for enhancing cognitive processes (56, 80).
In assessing the potential effectiveness of a treatment targeting cognitive processes, it is also important to consider that many of the processes and skills impaired after a TBI require routine use in order to maintain proficiency. Rehabilitation of damaged neural circuits entails repeated engagement of those circuits in a manner that is potent and that enables transfer from the target behavior to related behaviors (81). Neurostimulation can create an ideal environment to promote plastic changes, but active engagement is likely necessary to facilitate and sustain desired changes. Pink and colleagues (82) performed a scoping review examining 30 studies of rTMS and TBI. They noted that the treatment generally had minimal adverse effects and was associated with the reduction of both physiological and psychosocial sequelae of injury. Remediation of cognitive deficits was, however, more variable with a limited number of studies examining cognitive response to treatment. Nonetheless, pairing rTMS/iTBS with cognitive interventions may increase the clinical benefits of these interventions because it enables direct targeting of hampered processes in a neural environment made more responsive to change by rTMS/iTBS stimulation.
Cognitive Rehabilitation
CR is a multifaceted process intended to aid patients with successful engagement and completion of functional tasks by identifying, training, and implementing the use of accommodations to compensate for deficits in cognition resulting from injury or disease, while at times also targeting recovery of deficient cognitive processes. CR consists, in part, of participant education and skills training intended to aid recovery of function by remediating persisting cognitive and functional deficits (83). Given the impact of mental health symptoms on cognitive functioning, as well as the high comorbidity of mood symptoms and cognitive concerns post-TBI, many CR programs also integrate a mindfulness, stress management, or affective arousal regulation component. Some CR programs have even combined more formal, manualized empirically validated mental health treatments (e.g., cognitive processing therapy for PTSD) with traditional treatments that have demonstrated significant improvements in cognitive and functional outcomes (SmartCPT) (84, 85). Programs may also integrate motivational interviewing and structured goal setting.
Individuals participating in CR for TBI engage in compensatory strategy training (e.g., learning to use both internal and external memory aids, attentional or executive functioning strategies, and other tools to accommodate and compensate for deficits) with the intent of improving both cognitive and functional well-being. Individuals receive training in how to properly use accommodations and incorporate these strategies into their IADLs (e.g., managing medications and finances, cooking) with a goal of routine use enabling greater functional independence (Figure 3).
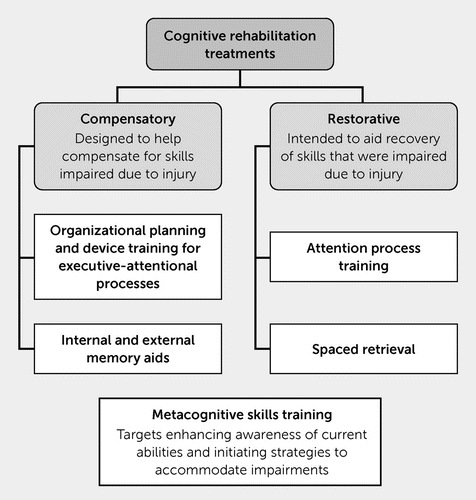
FIGURE 3. Cognitive rehabilitation treatmentsa
aSeveral examples of compensatory and restorative treatments that may be used during cognitive rehabilitation are provided to clarify how treatments differ. This list is not exhaustive. Compensatory strategy training is most commonly used during cognitive rehabilitation because restorative treatments have not been consistently proven to facilitate return to baseline.
Additionally, metacognitive skills training (e.g., targeting deficit awareness and functionally connecting how impairments may influence participation in IADLs) may also be a component of CR. A goal of this intervention is to enable individuals to identify barriers and navigate them by using knowledge they already have and accommodations and skills that may be developed during CR. Early systematic reviews of metacognitive interventions and treatment of cognitive impairments secondary to TBI have reported positive findings but noted that the number of studies available for analysis was limited (14). A recent meta-analysis of acquired brain injury (e.g., stroke, TBI) observed that metacognitive intervention is associated with better functional outcomes, but methodologies for using the treatment vary, highlighting the need for more research (86). One of the perceived benefits of metacognitive skills training is that it can be incorporated into a variety of treatment plans, which likely influences the success of the intervention.
CR programs may expand outside accommodations and include restorative exercises (e.g., attention training, spaced-memory exercises) or cognitive training exercises that are intended to directly enhance cognitive ability. An additional aim of restorative interventions is to aid recovery by inducing plastic changes within the brain. Similar to use of rTMS, CR is associated with changes in neural activation and structural connectivity influencing multiple neural networks (e.g., central executive network [CEN], default mode network [DMN], dorsal anterior network [DAN]), although there is a wide range of individual variability with respect to response to treatment (87–90). Additionally, empirical support on the ability to translate skills from these training activities to daily life is mixed (12, 13, 91). There are, however, sufficient positive findings providing efficacy for further research with current work, suggesting that TMS may bolster or strengthen the ability of restorative exercises to prompt functional changes in various cognitive domains.
Attention process training.
Attention is an area frequently targeted during cognitive intervention because it is commonly impaired after a TBI. Remediation of attentional deficits is a major challenge for clinicians and a key area of concern, particularly with respect to the safety of the patient and the patient’s family during IADLs (e.g., ensuring basic and sustained attention to task while cooking, ironing, or taking medication). Attention process training (APT) is a hierarchical training protocol intended to improve several types of attention (e.g., sustained, divided, alternating) with use of exercises that engage executive-attentional skills, including working memory and metacognitive skills (92–94). Several studies examined the effectiveness of earlier versions of APT (current version is APT-3, a computerized version of the treatment); results showed gains in individuals with a history of mild (95) and severe TBI (96), as well as in individuals who had a general history of TBI with persisting symptoms (97). Furthermore, a study assessing APT effectiveness in acquired brain injury (e.g., stroke and TBI) reported that early intervention was beneficial for participants in both groups, with improvements maintained at the 6-month follow-up (98). Collectively, these studies lend support for using APT at all stages of TBI recovery.
Attention training paired with rTMS/iTBS.
Studies of rTMS/iTBS and attention are still in their infancy, although there is burgeoning evidence that rTMS delivered to the DLPFC can enhance the executive-attentional processing of individuals with a history of TBI (22, 23). Studying healthy individuals by using a unique site of stimulation, Esterman et al. (99) reported that transient attention improves after iTBS delivered to either the midline cerebellar node of the DAN or the right cerebellar node of the DMN. However, sustained attention was improved only after stimulation of the DAN. Treatment approaches likely to yield the largest gain for attention are those that enable physiological change, while engaging skill sets that are pertinent to everyday tasks. Pairing rTMS/iTBS to DLPFC or nodes of the DAN and DMN with behavioral interventions such as APT may augment remediation of attentional deficits. Further research is needed in this area to aid understanding of these findings.
Memory training.
Deficits to explicit memory, particularly recall of facts and events (semantic and episodic memory) and acquisition of new information (encoding of episodic and semantic information), are common after a TBI. A common behavioral treatment for memory deficits is spaced retrieval (also termed the “spacing effect”). It consists of the presentation of or request for target information, with the target response produced repeatedly over increasingly longer time intervals. Spaced retrieval with persons with a history of TBI has produced positive outcomes, indicating that the technique can enhance memory and learning (100–102).
Memory training paired with rTMS/iTBS.
Examining TMS as a means of enhancing memory is also still in the earlier stages of development with studies just beginning to uncover the potential influence of rTMS on memory processes. Unlike much of the previous research discussed, site of stimulation for memory thus far has focused on the lateral parietal cortex (LPC), which is a component of the cortical hippocampal network. Wang et al. (103) examined whether 5 consecutive days of rTMS delivered to the LPC could enhance associative memory (a subtype of episodic memory) in healthy individuals. Relative to sham stimulation, rTMS improved memory tested 24 hours after the session. rTMS was also associated with positive changes in neural connectivity in the cortical hippocampal network, results that have now been replicated (104).
These findings suggest that iTBS may be used to strengthen the neural network and directly aid memory. Pairing memory strategies (such as spaced retrieval) with iTBS has the potential to strengthen neural circuits and produce lasting functional changes that would greatly benefit persons with residual symptoms from TBI. In the same manner that site of stimulation is key, use of ecologically valid cognitive assessments is critical to ensure that gains endure and are not limited to clinical or laboratory settings.
Paired Treatment of MDD
In treatment of co-occurring MDD with TBI, best practice aims to address the depressive symptomatology independent of etiology (e.g., as potentially related to history of TBI), using the same empirically validated psychosocial and behavioral interventions (e.g., cognitive-behavioral therapy, behavioral activation, acceptance and commitment therapy).
rTMS is currently most commonly recommended for use with treatment-resistant MDD (i.e., the treatment to use when other options fail to yield clinically significant gains) (105, 106). Paired TMS treatment and psychotherapy have an observed response rate of 66% and a remission rate of 56% (107). Work examining the use of rTMS as a treatment for MDD with comorbid TBI is limited but growing. Case studies have provided initial evidence that rTMS may facilitate a decrease in depressive symptoms for individuals with a history of mild TBI (108) or severe TBI (109). However, these findings may not be consistent across individuals. A randomized control trial (RCT) by Rao et al. (110) revealed a small effect of treatment for individuals with TBI with highly variable responses to treatment between participants. The authors noted that parameters of stimulation may influence treatment outcomes; their study used low-frequency stimulation to the right DLPFC. Additionally, they also remarked that time postinjury may have been a confounding variable influencing their outcomes. Finally, an RCT by Siddiqi et al. (38) found stimulation delivered to left DLPFC resulted in clinical gains that were beyond what was observed with sham stimulation.
There have been only a few RCTs that have examined how rTMS has influenced cognitive and psychosocial deficits after a TBI. Lee and Kim (22) examined rTMS and depression. They noted that treatment reduced depressive symptoms and improved cognitive functioning in specific aspects of executive functioning for the treatment group. Gains were not observed for participants in a control group. Hoy et al. (23) reported similar findings with the active treatment group displaying improved performance in aspects of cognitive functioning (i.e., executive functioning, working memory). As mentioned previously, participants in this study displayed improvement with respect to depressive symptoms, regardless of the treatment received (active or sham).
Paired Treatment of PTSD
In treating PTSD co-occurring with residual symptoms from TBI, it is pertinent to address emotional responses to stressors, while also targeting cognitive impairment. Psychological treatment of PTSD takes many forms including, but not limited to, empirically based treatments such as prolonged exposure (PE) (111) and cognitive processing therapy (CPT) (112).
PE involves, in part, presenting aversive stimuli to a person in a controlled safe environment and teaching skills to manage anxiety and stress. Over time, the implementation of these skills during repeated or prolonged exposure reduces the anxiety response altogether, thus decreasing the negative impact of anxiety on IADLs. Fryml and colleagues (113) performed a randomized, double-blind, active sham-control pilot study examining the influence of the paired use of rTMS and PE for PTSD. Results were promising: the active group showed a larger decrease in symptoms relative to those in the control group (114), although scores did not achieve the threshold of significance.
CPT, in part, targets the response to thoughts and emotions pertaining to the traumatic event (115, 116). Behavioral research shows that pairing CPT with cognitive strategy training (specifically CogSMART) produces greater gains among persons who have comorbid TBI history (SmartCPT) (84, 85). Kozel et al. (117) compared the effectiveness of rTMS delivered in isolation to rTMS paired with CPT. Participants were randomly assigned to either active or sham rTMS with CPT delivered after TMS sessions. Paired intervention resulted in greater gains at 1-, 3-, and 6-month follow-ups. Collectively, these findings further support the use of pairing behavioral interventions with TMS treatments in order to facilitate positive long-term neurobehavioral changes.
Considerations and Limitations
Responses to any form of intervention are likely to vary between individuals. TBIs can produce an array of deficits, and these must each be accounted for in all interventions, particularly when considering rTMS and iTBS. A number of factors can influence treatment outcomes, including time postinjury, comorbid conditions, and pharmacological interventions. It is pertinent that these be accounted for when assessing cognitive and behavioral responses. Additionally, rTMS is not suited for everyone. Individuals who have stents in their brain or neck, metallic implants in their ears or eyes, implanted electrode devices above the thoracic cavity, and those who have potentially been exposed to shrapnel are not candidates for treatment.
With respect to clinical outcomes, we must again emphasize that not all studies of MDD and rTMS (30, 118, 119) or TBI and rTMS have yielded clinically meaningful positive findings (75–77, 110), indicating that further work is needed to establish whether rTMS can be used routinely with this population. Differences in site of stimulation and intensity of stimulation are likely to have played a role in responsiveness to treatment and continue to require further investigation. With respect to client and patient care, critical areas to focus on include neurobehavioral profiles, heterogeneity with respect to injury type (e.g., diffuse axonal injury, coup-contrecoup injury), and reported symptoms. Additionally, it is important to examine the site and intensity of stimulation. We chose to focus on MDD and PTSD for this review, but there are other conditions that could co-occur that require special consideration (e.g., schizophrenia, bipolar disorder).
With respect to CR, effectiveness of TMS must be measured in terms of participation and functional engagement in IADLs. Studies commonly measure performance on the basis of neuropsychological assessments, which may not translate to functional skills and may assess skills that were not directly targeted in treatment.
The effects of neurostimulation on cognitive processing are just beginning to be uncovered. Future research may identify additional cortical sites of stimulation that more effectively facilitate cognitive-behavioral gains and better tailor treatment dosage. Furthermore, the long-term effects of rTMS and iTBS remain unclear, particularly with respect to executive-attentional processing and memory. Most current evidence is limited to short-term gains. Continued research is needed to elucidate these concerns.
Conclusions
Rehabilitation of cognitive-psychosocial deficits experienced by persons with a history of TBI and commonly co-occurring PTSD requires interventions sufficient to induce a shift in neural processing evidenced behaviorally by enhanced performance. Studies of rTMS and iTBS show that neurostimulation has the potential to alter neural networks in a manner that may benefit special populations, such as persons with a history of TBI. Targeting regions such as the DLPFC with rTMS and iTBS may engender a neural network that is malleable and subsequently capable of adaptive functional changes. The evidentiary framework summarized here supports the pairing of rTMS and iTBS with cognitive interventions. The framework is intended to be a steppingstone for future research; strategic pairings have the potential to augment long-term gains and better ensure generalization of rehabilitated and trained skills to everyday life. Further research is needed to continue refining treatment protocols to produce the most efficacious outcomes.
1. : Estimating the global incidence of traumatic brain injury. J Neurosurg 2019; 130:1080–1097Crossref, Google Scholar
2. : Incidence, risk factors and prevention of mild traumatic brain injury: results of the WHO Collaborating Centre Task Force on Mild Traumatic Brain Injury. J Rehabil Med 2004; 36:28–60Crossref, Medline, Google Scholar
3. : The neuropsychological outcomes of concussion: a systematic review of meta-analyses on the cognitive sequelae of mild traumatic brain injury. Neuropsychology 2014; 28:321–336Crossref, Medline, Google Scholar
4. : The association between post-concussion symptoms and health-related quality of life in patients with mild traumatic brain injury. Injury 2019; 50:1068–1074Crossref, Medline, Google Scholar
5. : Health-related quality of life after TBI: a systematic review of study design, instruments, measurement properties, and outcome. Popul Health Metrics 2015; 13:4Crossref, Medline, Google Scholar
6. : Rates of major depressive disorder and clinical outcomes following traumatic brain injury. JAMA 2010; 303:1938–1945Crossref, Medline, Google Scholar
7. : Axis I psychopathology in individuals with traumatic brain injury. J Head Trauma Rehabil 1998; 13:24–39Crossref, Medline, Google Scholar
8. : Psychiatric comorbidity following traumatic brain injury. Brain Injury 2007; 21:1321–1333Crossref, Medline, Google Scholar
9. : Cognitive functioning in major depression—a summary. Front Hum Neurosci 2009; 3:26.
10. : A meta-analysis of cognitive functioning in older adults with PTSD. J Anxiety Disord 2013; 27:550–558Crossref, Medline, Google Scholar
11. : A quantitative meta-analysis of neurocognitive functioning in posttraumatic stress disorder. Psychol Bull 2015; 141:105–140Crossref, Medline, Google Scholar
12. : Evidence-based cognitive rehabilitation: systematic review of the literature from 2009 through 2014. Arch Phys Med Rehabil 2019; 100:1515–1533Crossref, Medline, Google Scholar
13. : Efficacy of memory rehabilitation therapy: a meta-analysis of TBI and stroke cognitive rehabilitation literature. Brain Injury 2014; 28:1610–1616Crossref, Medline, Google Scholar
14. : Intervention for executive functions after traumatic brain injury: a systematic review, meta-analysis and clinical recommendations. Neuropsychol Rehabil 2008; 18:257–299Crossref, Medline, Google Scholar
15. : Effectiveness of cognitive rehabilitation following acquired brain injury: a meta-analytic re-examination of Cicerone et al.’s (2000, 2005) systematic reviews. Neuropsychology 2009; 23:20–39Crossref, Medline, Google Scholar
16. : Cognitive rehabilitation for adults with traumatic brain injury to improve occupational outcomes. Cochrane Database Syst Rev 2017; 6:CD007935Google Scholar
17. : The Clinical TMS Society consensus review and treatment recommendations for TMS therapy for major depressive disorder. Brain Stimul 2016; 9:336–346Crossref, Medline, Google Scholar
18. FDA permits marketing of transcranial magnetic stimulation for treatment of obsessive compulsive disorder. Silver Spring, MD, US Food & Drug Administration, 2018. www.fda.gov/news-events/press-announcements/fda-permits-marketing-transcranial-magnetic-stimulation-treatment-obsessive-compulsive-disorder. Accessed Aug 22, 2020Google Scholar
19. : FDA clears first brain stimulation device to help smokers quit. Medscape 2020. www.medscape.com/viewarticle/936234. Accessed Oct 23, 2020Google Scholar
20. : Repetitive transcranial magnetic stimulation-associated neurobehavioral gains during coma recovery. Brain Stimul 2009; 2:22–35Crossref, Medline, Google Scholar
21. : Effects of 10 Hz repetitive transcranial magnetic stimulation of the left dorsolateral prefrontal cortex in disorders of consciousness. Front Neurol 2017; 8:182Crossref, Medline, Google Scholar
22. : Effect of low frequency repetitive transcranial magnetic stimulation on depression and cognition of patients with traumatic brain injury: a randomized controlled trial. Med Sci Monit 2018; 24:8789–8794Crossref, Medline, Google Scholar
23. : A pilot investigation of repetitive transcranial magnetic stimulation for post-traumatic brain injury depression: safety, tolerability, and efficacy. J Neurotrauma 2019; 36:2092–2098Crossref, Medline, Google Scholar
24. : The neurometabolic cascade of concussion. J Athl Train 2001; 36:228–235Medline, Google Scholar
25. : Traumatic axonal injury in patients with mild traumatic brain injury; in Advanced Diagnostics and Acute Management. Edited by Gorbunov NV, Long JB. InTech, 2018. www.intechopen.com/books/traumatic-brain-injury-pathobiology-advanced-diagnostics-and-acute-management/traumatic-axonal-injury-in-patients-with-mild-traumatic-brain-injury. Accessed Jul 23, 2020Google Scholar
26. : Axonal damage in traumatic brain injury. Neuroscientist 2000; 6:483–495Crossref, Google Scholar
27. : Network dysfunction after traumatic brain injury. Nat Rev Neurol 2014; 10:156–166Crossref, Medline, Google Scholar
28. : Disrupted intrinsic connectivity among default, dorsal attention, and frontoparietal control networks in individuals with chronic traumatic brain injury. J Int Neuropsychol Soc 2016; 22:263–279Crossref, Medline, Google Scholar
29. : Acute left prefrontal transcranial magnetic stimulation in depressed patients is associated with immediately increased activity in prefrontal cortical as well as subcortical regions. Biol Psychiatry 2004; 55:882–890Crossref, Medline, Google Scholar
30. : High (15 Hz) and low (1 Hz) frequency transcranial magnetic stimulation have different acute effects on regional cerebral blood flow in depressed patients. Psychol Med 2003;33:997–1006Crossref, Medline, Google Scholar
31. : Network-wise cerebral blood flow redistribution after 20 Hz rTMS on left dorso-lateral prefrontal cortex. Eur J Radiol 2018; 101:144–148Crossref, Medline, Google Scholar
32. : Repetitive transcranial magnetic stimulation promotes neural stem cell proliferation and differentiation after intracerebral hemorrhage in mice. Cell Transpl 2019; 28:568–584Crossref, Medline, Google Scholar
33. : rTMS of the left dorsolateral prefrontal cortex modulates dopamine release in the ipsilateral anterior cingulate cortex and orbitofrontal cortex. PLoS One 2009; 4:e6725Crossref, Medline, Google Scholar
34. : Long-term repetitive transcranial magnetic stimulation increases the expression of brain-derived neurotrophic factor and cholecystokinin mRNA, but not neuropeptide tyrosine mRNA in specific areas of rat brain. Neuropsychopharmacology 2000; 23:205–215Crossref, Medline, Google Scholar
35. : rTMS induces analgesia and modulates neuroinflammation and neuroplasticity in neuropathic pain model rats. Brain Res 2021; 1762:147427Crossref, Medline, Google Scholar
36. : Transcranial magnetic stimulation: a possible treatment for TBI. J Head Trauma Rehabil 2006; 21:437–451Crossref, Medline, Google Scholar
37. : Transcranial magnetic stimulation and environmental enrichment enhances cortical excitability and functional outcomes after traumatic brain injury. Brain Stimul 2018; 11:1306–1313Crossref, Medline, Google Scholar
38. : Repetitive transcranial magnetic stimulation with resting-state network targeting for treatment-resistant depression in traumatic brain injury: a randomized, controlled, double-blinded pilot study. J Neurotrauma 2019; 36:1361–1374Crossref, Medline, Google Scholar
39. : Inflammatory cytokines in depression: neurobiological mechanisms and therapeutic implications. Neuroscience 2013; 246:199–229Crossref, Medline, Google Scholar
40. : Depression: an inflammatory illness?. J Neurol Neurosurg Psychiatry 2012; 83:495–502Crossref, Medline, Google Scholar
41. : Inflammation mediates varying effects in neurogenesis: relevance to the pathogenesis of brain injury and neurodegenerative disorders. J Neurochem 2009; 108:1343–1359Crossref, Medline, Google Scholar
42. : The duality of the inflammatory response to traumatic brain injury. Mol Neurobiol 2001; 24:169–181Crossref, Medline, Google Scholar
43. : Glutamate and GABA imbalance following traumatic brain injury. Curr Neurol Neurosci Rep 2015; 15:27Crossref, Medline, Google Scholar
44. : Ventromedial prefrontal cortex/anterior cingulate cortex Glx, glutamate, and GABA levels in medication-free major depressive disorder. Transl Psychiatry 2021; 11:419Crossref, Medline, Google Scholar
45. : Acute inflammatory biomarker profiles predict depression risk following moderate to severe traumatic brain injury. J Head Trauma Rehabil 2015; 30:207–218Crossref, Medline, Google Scholar
46. : Inflammation in post-traumatic stress disorder (PTSD): a review of potential correlates of PTSD with a neurological perspective. Antioxidants 2020; 9:107Crossref, Medline, Google Scholar
47. : Comorbidity between post-traumatic stress disorder and major depressive disorder: alternative explanations and treatment considerations. Dialogues Clin Neurosci 2015; 17:141–150Crossref, Medline, Google Scholar
48. : Effect of rTMS on GABA and glutamate levels in treatment-resistant depression: an MR spectroscopy study. Psychiatry Res Neuroimaging 2021; 317:111377Crossref, Medline, Google Scholar
49. : Transcranial magnetic stimulation: a primer. Neuron 2007; 55:187–199Crossref, Medline, Google Scholar
50. : Transcranial magnetic stimulation and the human brain. Nature 2000; 406:147–150Crossref, Medline, Google Scholar
51. : Responses to rapid-rate transcranial magnetic stimulation of the human motor cortex. Brain 1994; 117:847–858Crossref, Medline, Google Scholar
52. : Cerebral blood flow and cognitive functioning in a community-based, multi-ethnic cohort: the SABRE Study. Front Aging Neurosci 2018; 10:279Crossref, Medline, Google Scholar
53. : Acute prefrontal rTMS increases striatal dopamine to a similar degree as d-amphetamine. Psychiatry Res Neuroimaging 2007; 156:251–255Crossref, Google Scholar
54. : Functional connectivity of the left DLPFC to striatum predicts treatment response of depression to TMS. Brain Stimul 2017; 10:919–925Crossref, Medline, Google Scholar
55. : Optimizing functional accuracy of TMS in cognitive studies: a comparison of methods. J Cogn Neurosci 2009; 21:207–221Crossref, Medline, Google Scholar
56. : Customizing TMS applications in traumatic brain injury using neuroimaging. J Head Trauma Rehabil 2020; 35:401–411Crossref, Medline, Google Scholar
57. : Using neuroimaging to individualize TMS treatment for depression: toward a new paradigm for imaging-guided intervention. NeuroImage 2017; 148:1–7Crossref, Medline, Google Scholar
58. : Repetitive transcranial magnetic stimulation for treatment-resistant depression: recent critical advances in patient care. Curr Treat Options Psych 2021; 8:47–63Crossref, Medline, Google Scholar
59. : Efficacy of repetitive transcranial magnetic stimulation using a figure-8-coil or an H1-Coil in treatment of major depressive disorder; a randomized clinical trial. J Psychiatry Res 2019; 114:113–119Crossref, Medline, Google Scholar
60. : rTMS of the dorsomedial prefrontal cortex for major depression: safety, tolerability, effectiveness, and outcome predictors for 10 Hz versus intermittent theta-burst stimulation. Brain Stimul 2015; 8:208–215Crossref, Medline, Google Scholar
61. : Theta burst stimulation of the human motor cortex. Neuron 2005; 45:201–206Crossref, Medline, Google Scholar
62. : The theoretical model of theta burst form of repetitive transcranial magnetic stimulation. Clin Neurophysiol 2011; 122:1011–1018Crossref, Medline, Google Scholar
63. : Transcranial magnetic stimulation: new insights into representational cortical plasticity. Exp Brain Res 2003; 148:1–16Crossref, Medline, Google Scholar
64. : Basic principles of transcranial magnetic stimulation (TMS) and repetitive TMS (rTMS). Ann Phys Rehabil Med 2015; 58:208–213Crossref, Medline, Google Scholar
65. : Transcranial magnetic stimulation, synaptic plasticity and network oscillations. J Neuroengineering Rehabil 2009; 6:7Crossref, Medline, Google Scholar
66. : Synaptic plasticity and memory: an evaluation of the hypothesis. Annu Rev Neurosci 2000; 23:649–711Crossref, Medline, Google Scholar
67. : Long-term potentiation and long-term depression: a clinical perspective. Clinics 2011; 66:3–17Crossref, Medline, Google Scholar
68. : Theta oscillations in the hippocampus. Neuron 2002; 33:325–340Crossref, Medline, Google Scholar
69. : Efficacy and time course of theta burst stimulation in healthy humans. Brain Stimul 2015; 8:685–692Crossref, Medline, Google Scholar
70. : Motor cortex plasticity predicts recovery in acute stroke. Cereb Cortex 2010; 20:1523–1528Crossref, Medline, Google Scholar
71. : Implementation of intermittent theta burst stimulation compared to conventional repetitive transcranial magnetic stimulation in patients with treatment resistant depression: a cost analysis. PLoS One 2019; 14:e0222546Crossref, Medline, Google Scholar
72. : High-dose spaced theta-burst TMS as a rapid-acting antidepressant in highly refractory depression. Brain 2018; 141:e18Crossref, Medline, Google Scholar
73. : Effectiveness of theta burst versus high-frequency repetitive transcranial magnetic stimulation in patients with depression (THREE-D): a randomised non-inferiority trial. Lancet 2018; 391:1683–1692Crossref, Medline, Google Scholar
74. : Transcranial magnetic stimulation (TMS) safety with respect to seizures: a literature review. Neuropsychiatr Dis Treat 2020; 16:2989–3000Crossref, Medline, Google Scholar
75. : No effects of 20 Hz-rTMS of the primary motor cortex in vegetative state: a randomised, sham-controlled study. Cortex 2015; 71:368–376Crossref, Medline, Google Scholar
76. : Effects of 20 hz repetitive transcranial magnetic stimulation on disorders of consciousness: a resting-state electroencephalography study. Neural Plasticity 2018; 2018:1–8Crossref, Google Scholar
77. : Effect of high-frequency repetitive transcranial magnetic stimulation on brain excitability in severely brain-injured patients in minimally conscious or vegetative state. Brain Stimul 2013; 6:913–921Crossref, Medline, Google Scholar
78. : Repetitive TMS does not improve cognition in patients with TBI: a randomized double-blind trial. Neurology 2019; 93:e190–e199Crossref, Medline, Google Scholar
79. : Noninvasive brain stimulation for persistent postconcussion symptoms in mild traumatic brain injury. J Neurotrauma 2015; 32:38–44Crossref, Medline, Google Scholar
80. : Transcranial magnetic stimulation: potential treatment for co-occurring alcohol, traumatic brain injury and posttraumatic stress disorders. Neural Regen Res 2014; 9:1712Crossref, Medline, Google Scholar
81. : Principles of experience-dependent neural plasticity: implications for rehabilitation after brain damage. J Speech Lang Hear Res 2008; 51:S225–S239Crossref, Medline, Google Scholar
82. : The use of repetitive transcranial magnetic stimulation (rTMS) following traumatic brain injury (TBI): a scoping review. Neuropsychological Rehabil 2021; 31:479–505Crossref, Medline, Google Scholar
83. : Optimizing Cognitive Rehabilitation Effective Instructional Methods. New York, Guilford Press, 2011Google Scholar
84. : Evaluation of a hybrid treatment for veterans with comorbid traumatic brain injury and posttraumatic stress disorder: study protocol for a randomized controlled trial. Contemp Clin Trials 2015; 45:210–216Crossref, Medline, Google Scholar
85. : SMART-CPT for veterans with comorbid post-traumatic stress disorder and history of traumatic brain injury: a randomised controlled trial. J Neurol Neurosurg Psychiatry 2019; 90:333–341Crossref, Medline, Google Scholar
86. : Metacognitive knowledge and functional outcomes in adults with acquired brain injury: a meta-analysis. Neuropsychological Rehabil 2021; 31:453–478Crossref, Medline, Google Scholar
87. : Training of goal-directed attention regulation enhances control over neural processing for individuals with brain injury. Brain 2011; 134:1541–1554Crossref, Medline, Google Scholar
88. : Investigating the neurobiological basis of cognitive rehabilitation therapy with fMRI. Brain Injury 2004; 18:957–974Crossref, Medline, Google Scholar
89. : Plasticity of the attentional network after brain injury and cognitive rehabilitation. Neurorehabil Neural Repair 2009; 23:468–477Crossref, Medline, Google Scholar
90. : Intensive cognitive rehabilitation therapy for chronic traumatic brain injury: a case study of neural correlates of functional improvement. Aphasiology 2019; 33:289–319Crossref, Google Scholar
91. : Do “brain-training” programs work? Psychol Sci Public Interest 2016; 17:103–186Crossref, Medline, Google Scholar
92. : Attention Process Training II: A Program to Address Attentional Deficits for Persons With Mild Cognitive Dysfunction. Puyallup, WA, Association for Neuropsychological Research and Development, 2001Google Scholar
93. : Attention Process Training-3. Youngsville, NC, Lash & Associates Publishing/Training, 2010Google Scholar
94. : Effectiveness of an attention-training program. J Clin Exp Neuropsychol 1987; 9:117–130Crossref, Medline, Google Scholar
95. : The rehabilitation of attention in individuals with mild traumatic brain injury, using the APT-II programme. Brain Injury 2000; 14:535–548Crossref, Medline, Google Scholar
96. : Rehabilitation of attention in two patients with traumatic brain injury by means of “attention process training.” Brain Injury 2006; 20:1207–1219Crossref, Medline, Google Scholar
97. : Evaluation of attention process training and brain injury education in persons with acquired brain injury. J Clin Exp Neuropsychol 2000; 22:656–676Crossref, Medline, Google Scholar
98. : Beneficial effects of early attention process training after acquired brain injury: a randomized controlled trial. J Rehabil Med 2020; 52:1–9Crossref, Google Scholar
99. : Network-targeted cerebellar transcranial magnetic stimulation improves attentional control. NeuroImage 2017; 156:190–198Crossref, Medline, Google Scholar
100. : Changes in activity participation following traumatic brain injury. Neuropsychological Rehabil 2017; 27:472–485Crossref, Medline, Google Scholar
101. : Spacing of repetitions improves learning and memory after moderate and severe TBI. J Clin Exp Neuropsychol 2003; 25:49–58Crossref, Medline, Google Scholar
102. : The effects of cognitive teletherapy on reported everyday memory behaviours of persons with chronic traumatic brain injury. Brain Injury 2007; 21:1245–1257Crossref, Medline, Google Scholar
103. : Targeted enhancement of cortical-hippocampal brain networks and associative memory. Science 2014; 345:1054–1057Crossref, Medline, Google Scholar
104. : Episodic memory improvements due to noninvasive stimulation targeting the cortical-hippocampal network: a replication and extension experiment. Brain Behav 2019; 9:e01393Crossref, Medline, Google Scholar
105. : American Psychiatric Association practice guidelines for the treatment of patients with major depressive disorder. Am J Psychiatry 2010; 167:9–118Google Scholar
106. : Therapeutic options for treatment-resistant depression. CNS Drugs 2010; 24:131–161Crossref, Medline, Google Scholar
107. : Simultaneous rTMS and psychotherapy in major depressive disorder: clinical outcomes and predictors from a large naturalistic study. Brain Stimul 2018; 11:337–345Crossref, Medline, Google Scholar
108. : Transcranial magnetic stimulation for depression after a traumatic brain injury: a case study. J ECT 2011; 27:38–40Crossref, Medline, Google Scholar
109. : Repetitive transcranial magnetic stimulation for treatment of depression in a patient with severe traumatic brain injury. TOJ 2018; 18:264–267Crossref, Google Scholar
110. : Low-frequency right repetitive transcranial magnetic stimulation for the treatment of depression after traumatic brain injury: a randomized sham-controlled pilot study. J Neuropsychiatry Clin Neurosci 2019; 31:306–318Link, Google Scholar
111. : Psychosocial treatments for posttraumatic stress disorder: a critical review. Annu Rev Psychol 1997; 48:449–480Crossref, Medline, Google Scholar
112. : Cognitive Processing Therapy for PTSD: A Comprehensive Manual, New York, Guilford Publications, 2016Google Scholar
113. : Exposure therapy and simultaneous repetitive transcranial magnetic stimulation: a controlled pilot trial for the treatment of posttraumatic stress disorder. J ECT 2019; 35:53–60Crossref, Medline, Google Scholar
114. : The development of a clinician-administered PTSD scale. J Traum Stress 1995; 8:75–90Crossref, Medline, Google Scholar
115. : Cognitive processing therapy for veterans with military-related posttraumatic stress disorder. J Consulting Clin Psychol 2006; 74:898–907Crossref, Medline, Google Scholar
116. : Cognitive processing therapy for sexual assault victims. J Consulting Clin Psychol 1992; 60:748–756Crossref, Medline, Google Scholar
117. : Repetitive TMS to augment cognitive processing therapy in combat veterans of recent conflicts with PTSD: a randomized clinical trial. J Affective Disord 2018; 229:506–514Crossref, Medline, Google Scholar
118. : Lack of a therapeutic effect of a 2-week sub-threshold transcranial magnetic stimulation course for treatment-resistant depression. Psychiatry Res 2002; 113:245–254Crossref, Medline, Google Scholar
119. : Repetitive transcranial magnetic stimulation (rTMS) in pharmacotherapy-refractory major depression: comparative study of fast, slow and sham rTMS. Psychiatry Res 1999; 88:163–171Crossref, Medline, Google Scholar