Age-Related Changes in Brain Glucose Metabolism in Adults With Attention-Deficit/Hyperactivity Disorder and Control Subjects
Abstract
Using positron emission tomography and [18F]-2-fluoro-2-deoxy-d-glucose, the authors determined cerebral metabolic rates for glucose (CMRglc) in 39 adults (18–51 years old) with attention-deficit/hyperactivity disorder (ADHD) and 56 healthy control adults (19–56 years old) during the performance of a continuous attention task. Increased age was associated with reduced global CMRglc in ADHD women, but not in ADHD men, control men, or control women. Better performance on the attention task was significantly associated with increased age only in the ADHD female group. Determining the role of behavioral, hormonal, and genetic factors is a challenge for future research.
Age-related changes in cerebral metabolic rate for glucose (CMRglc) and cerebral blood flow in normal adults have been studied for the past 40 years. Table 1 indicates that findings using various methodologies (Kety-Schmidt method; Xenon SPECT; PET) showed either no relationship or reduction of brain glucose metabolism and cerebral blood flow with increased age. The age range in these studies often extended into the geriatric period, and the number of studies reporting no age effect might have been larger if elderly subjects had been excluded. The one study that did not include elderly individuals reported no effect of age on brain glucose metabolism.1 The significance of age-related changes of brain activity not only pertains to the understanding of aging, but also represents a potential means to investigate neural substrates of psychiatric disorders. Studying differential influence of age on CMRglc as a function of psychiatric disorder may clarify underlying mechanisms of the course and etiology of psychopathology.
This study tests the hypothesis that age-related changes of global CMRglc are greater in adults with attention-deficit/hyperactivity disorder (ADHD) compared with healthy control volunteers. This assumption is based on previous work showing that ADHD adults, and not ADHD adolescents, had abnormally low CMRglc, suggesting an age effect of ADHD on brain glucose metabolism.2,3 In addition, ADHD females tended to have more deviant glucose metabolic rates than ADHD males, both in adults (CMRglc lower in ADHD compared with control subjects, by 12.7% in women and by 6.0% in men)3 and in adolescents (CMRglc lower in ADHD compared with control subjects, by 15% in girls and 0% in boys).4 This difference implies a greater impact of ADHD on brain glucose metabolism in females than in males.4 The present report is restricted mainly to the influence of age on overall cortical glucose metabolism.
METHODS
Subjects
Data were collected from 95 adult subjects, ages 18 to 56 years, of whom 39 had ADHD and 56 were healthy control volunteers. Healthy volunteers were recruited through advertisements in local newspapers and through the National Institutes of Health office of normal volunteers. The ADHD adults were recruited also through advertisements in local newspapers and an ADHD advocacy and support organization. These subjects participated in studies of CMRglc performed under identical conditions between August 1988 and May 1992.1,5–7 Written informed consent was obtained for all subjects after the procedure had been fully explained.
All subjects had a complete physical and laboratory medical workup. They were evaluated psychiatrically by the Schedule for Affective Disorders and Schizophrenia (lifetime version).8 Control subjects were free of any Axis I psychiatric disorders. Adults with ADHD met exclusively current and childhood (retrospectively) DSM-III/DSM-III-R criteria for ADHD, as well as Utah criteria.9 The diagnosis was made by a psychiatrist board certified in child and adult psychiatry with extensive experience in ADHD (A.J.Z.). The Utah criteria are designed for the diagnosis of ADHD specifically in adults. Whenever possible, school records were obtained, and the 10-item Conners rating scale10 was completed by the parents.
Exclusion criteria were the presence of any other Axis I psychiatric disorders, other than ADHD, and of any medical problems, neurological disorders, or history of head trauma with loss of consciousness. Subjects had to be free of all medications for at least 3 weeks prior to the scan.
Procedures
Prior to the PET study, all subjects were trained on a continuous auditory discrimination attention task (CPT).11 The CPT provided an index of attentional skill, which is the core deficit in ADHD. Subjects were asked to press a button each time they heard the lowest intensity tone (67 dB) of 3 tones (500 Hz). The tones lasted 1 second and were presented at 2-second intervals in random order. The sequence of the 3 randomized tones was identical for all subjects. Subjects performed this task with eyes patched during the 30-minute uptake of the tracer [18F]-2-fluoro-2-deoxy-d-glucose (18-FDG). Two variables were analyzed: the number of correctly identified targets (HITS), and the number of distractors misidentified as targets (false alarms, FA). Accuracy of performance was defined as log(HITS/[FA+1]).5
The tracer 18-FDG was infused intravenously over 1 minute at a dose of 5 mCi. Within 5 minutes after the end of the scanning period, the Spielberger State-Trait Anxiety Inventory (STAI)12 was completed for the 30-minute period of tracer uptake to control for the potential influence of anxiety on CMRglc.13,14
A 7-slice brain Scanditronix scanner was used. The in-plane resolution was 5.2 mm, and the axial resolution was 10 mm. Four sets of slices (28 images), starting at 5 mm above a plane parallel to the canthomeatal line (CM), were obtained, with an interslice interval of approximately 3.5 mm. Transmission scans were used to correct for radiation attenuation through skull and brain tissue.15 Tracer input curves were calculated from arterial blood samples drawn through a radial arterial line.
Raw pixel values were converted to glucose metabolic rates in milligrams of glucose per 100 g of tissue per minute.16,17 Regional rates of glucose metabolism (rCMRglc) were analyzed by an independent rater, blind to diagnosis, for 60 regions of interest (ROIs) distributed within 5 standard transaxial planes (planes A–E). The most superior plane, plane A, included bilateral white matter structures corresponding to a horizontal section of the corona radiata above the level of the corpus callosum. Plane B was also above the body of the corpus callosum and contained the middle cingulate region and bilateral white matter strips. Plane C comprised the body of the corpus callosum. Plane D was characterized by the presence of the basal ganglia, the internal capsule, and the thalamus. The lowest plane, plane E, included the lower frontal and temporal lobes and the hippocampal gyrus. These planes are represented in Figure 1. The regions of interest (2.9 cm2 rectangular boxes of 49 pixels each) were placed on each subject's image, using a standard template based on the human brain atlas of Matsui and Hirano.18 Global CMRglc was calculated as the average rate of all the gray matter–rich areas of the cortex examined (average of 52 cortical regions of interest of the 60 cortical and subcortical regions sampled).
Statistical Analysis
Because of a drift in measurements over time (higher values of CMRglc with later date of scan: r=0.40, n=95, P=0.0001), statistical analyses were performed on the residuals of CMRglc values regressed on date of scan.
Two-way analyses of variance (ANOVAs) with two between-group factors, gender (male/female) and diagnosis (ADHD/control), were used to compare groups on age, education, anxiety state, CPT performance, and global CMRglc. The demographic and clinical variables that differed significantly among groups were correlated with global CMRglc and age to assess the need for controlling these variables in subsequent analyses.
An overall ANOVA (regression analysis across groups using the General Linear Models [GLM] procedure from the SAS program) was used to evaluate the slopes and intercepts of regression lines of [global CMRglc×age] in all 4 groups (ADHD women, ADHD men, control women, control men). For a statistically significant overall ANOVA, post hoc regression analyses assessed whether individual regression lines were different from zero in each group, and whether they differed from each other.
For the sake of completeness, correlations between regional CMRglc and age were computed for each group by Pearson correlation coefficient analyses, and the Bonferroni correction factor was applied to correct for multiple tests (60 tests per group). An alpha value lower than 0.0008 (0.05/60) indicated statistical significance at P<0.05. Absolute and normalized (regional/global) regional CMRglc values (rCMRglc) were used. Normalized rCMRglc are commonly employed to control for the influence of interindividual differences in global CMRglc on regional CMRglc.
RESULTS
Sample Characteristics
The characteristics of the sample are presented in Table 2. Gender distribution was similar in the control and ADHD groups (χ2=0.44, df=1, not significant). By two-way ANOVA, there was no significant gender×diagnosis interaction with respect to education, age, anxiety state (STAI rating scores), or CPT performance. Diagnosis and gender had no significant main effects on levels of education (diagnosis: F=0.05, df=1,91, not significant; gender: F=0.27, df=1,91, not significant). However, there was a significant main effect of diagnosis, and not gender, for age, anxiety state, and CPT performance (HITS). Although the age range in the control group (19.3 to 56.3 years) was greater than in the ADHD group (18.1 to 50.8 years), the group of control adults (n=56; 28.6±7.5 years old) was significantly younger than the ADHD group (n=39; 35.2±9.9 years old; F=12.79, df=1,91, P<0.001). Performance on the auditory attention task (HITS and accuracy) was significantly worse in the ADHD group compared with the control group (HITS: F=4.56, df=1,77 P=0.018; Accuracy: F=3.68, df=1,77, P=0.030). Finally, STAI anxiety scores during the uptake of the tracer were higher in the ADHD group (37.8±9.2) than in the control group (31.5±8.6; F=12.63, df=1,90, P<0.001), but not different between women and men in either group.
Cerebral Glucose Metabolism (Table 3)
Within the whole sample, CPT-HITS, CPT-Accuracy and STAI anxiety scores did not correlate with global CMRglc (n=95, r=−0.15, −0.05, −0.10, respectively) or with age (n=95, r=−0.06, −0.18, −0.16, respectively), and they were not controlled for subsequent analyses. By group, CPT-HITS was correlated with age only in the ADHD women, where older women performed better (r=0.67, n=13, P=0.013; Figure 2). Anxiety scores on the STAI did not correlate with age or CMRglc in any of the four groups.
By two-way ANOVA, a diagnosis×gender interaction was not found for global CMRglc. The main effect of diagnosis was not significant (F=0.03, df=1,91, not significant), but there was a significant effect of gender (F=10.65, df=1,91, P<0.002). Global CMRglc was higher in women than in men (t=3.41, df=93, P=0.001).
The overall regression analysis (GLM-SAS) of age with global CMRglc was statistically significant (F=3.35, df=8,87, P=0.002), indicating that regression lines were different from each other among groups. Post hoc tests showed that only ADHD women had a regression line of age * global metabolism different from zero (r=–0.74; n=15; P=0.002; Figure 3), and none of the other 3 groups. This test was still significant when CPT-HITS was partialled out (r=–0.68, n=13, P=0.014). The regression line of age * global CMRglc of the ADHD women was significantly different from that of the ADHD men (F=7.34, df=2,35, P=0.002), the control men (F=5.61, df=2,41, P=0.007), and the control women (F=4.9, df=2,37, P=0.013). The relationships between age and global CMRglc are illustrated in Figure 3 for the ADHD group and Figure 4 for the control group. When the correction for the drift in measurement with time was not applied, the statistical significance of the correlations between age and global CMRglc remained statistically significant only for the ADHD women.
For the sake of completeness, Table 4 and Table 5 show the Pearson correlation coefficients of the absolute and normalized rCMRglc with age in the four groups. After Bonferroni correction for multiple statistical tests, none of the correlations of either absolute or normalized rCMRglc values reached significance in the male control or female control groups. In women with ADHD, 18 of the 60 ROIs sampled (30%) showed significant correlations (all negative) of absolute rCMRglc rates with age, reflecting the influence of age on global CMRglc. In ADHD men, a single region, the left anterior frontal cortical area in plane D, showed a significant correlation between absolute rCMRglc and age (r=0.54, n=24, P=0.0008). For normalized rCMRglc values (regional/global), significant correlations with age were found in the anterior medial frontal cortex in plane B in the group of ADHD women (r=0.79, n=15, P=0.0008) and in the left and right anterior frontal cortices of plane D in the group of ADHD men (left:r=0.68, n=24, P=0.0003; right: r=0.67, n=24, P=0.0005). These correlations will not be discussed given the lack of a priori hypotheses.
Because of the age difference between groups, the data analysis was repeated on a smaller sample (20 subjects removed blindly in regard to subjects' characteristics, except their age), but with groups matched by age (groups on age: F=0.43, df=3,71, P=0.7). Findings were similar to those obtained from the full sample (data available on request).
DISCUSSION
Increased age from the second to the sixth decades of life (between 18 and 56 years) was associated with a reduction of global CMRglc in women with ADHD, but not in control women, ADHD men, or control men. In the ADHD female group, performance on the CPT was not correlated with global CMRglc, although it was correlated with age. These findings suggest the influence of age on both attentional skill and CMRglc selectively in ADHD women. There is anecdotal evidence for clinical improvement of ADHD symptoms with age.19 Adult patients commonly report having compensated for their attentional deficits and being less hyperactive with age. Decreased regional brain activity and improved performance may reflect increased efficiency of the brain to perform a given task.20 Although unlikely because of the age-related improvement on CPT performance, decreased global CMRglc with age could also indicate neuronal loss.
As expected, CPT performance was lower in the ADHD group compared with the control group. It is conceivable that the cognitive strategy or burden of mental effort associated with performing the CPT task changed with age differentially in ADHD women and the other groups. Esposito et al.21 reported gender-related differences in global cerebral blood flow (CBF) as a function of the nature of the cognitive task performed during scanning. This study suggested the influence of interactions between cognitive state and gender on brain activity.
Sex hormones may also contribute to gender- and age-related changes of brain activity. Gender differences in CBF seem to diminish after the fifth or sixth decade, when most women have reduced sex hormones from menopause.22–24 None of the ADHD women in the present study had entered menopause; the oldest ADHD woman was 47 years old. An interaction between ADHD, sex hormones, and brain activity is still possible, but it could not be tested in this study (data on menstrual cycle and hormonal levels unavailable). Future studies will need to consider all three factors—ADHD, cognitive state, and hormonal state—individually and interactively because 1) cognitive and motor impairments define ADHD;25 2) ADHD symptoms are gender related (lower incidence, less severe hyperactivity, and more severe cognitive deficits in girls than in boys);26–37 and 3) menstrual cycles (sex hormones) can influence performance of motor and perceptual-spatial tasks in control women.38
Lastly, two comments are in order on the effects of gender and diagnosis on CMRglc. Consistent with most PET studies,1,39,40 but not all,41,42 women showed higher global CMRglc than men in both ADHD and control groups throughout the age range, and this difference was significant throughout the age range in younger as well as in older subjects (data not shown). Global CMRglc (controlled for date of scan) was not different (mean CMRglc =[–0.013±1.7] mg˙100 g–1˙min–1) from that in the control group (mean CMRglc =[+0.010±1.9] mg˙100 g–1˙min–1). This lack of difference, which is in contrast to a previous report,3 may reflect higher variance of the data due to the post hoc nature of the present study.
A general caveat for studies of age effects concerns the use of a cross-sectional design. Such a design increases the variance of CMRglc and may not be powerful enough to detect the influence of age in all groups. Conversely, the significant association between age and global CMRglc in women with ADHD may be a sampling artifact and thus requires replication. The present findings emphasize the need to systematically study female subjects with ADHD and to control for or exploit the age effect in such research. The pathophysiology that underlies the age-related decrease of CMRglc selectively in this group may have great relevance to the understanding of ADHD pathogenesis. Given the evidence of a genetic influence and gender-related clinical differences in ADHD, the role of behavioral hormonal and genetic factors in brain abnormalities such as the one identified in the present work are challenges for future research.43 Finally, because not all children continue to meet ADHD criteria in adulthood,44–46 the study of adults with persistent ADHD raises the question of the neural substrates that contribute to the maintenance of the disorder into adulthood.
ACKNOWLEDGMENTS
The authors thank Lars Hungerford for his help in preparing the manuscript. Part of this work was presented as a poster at the Society of Nuclear Medicine annual meeting, Denver, CO, June 1996.
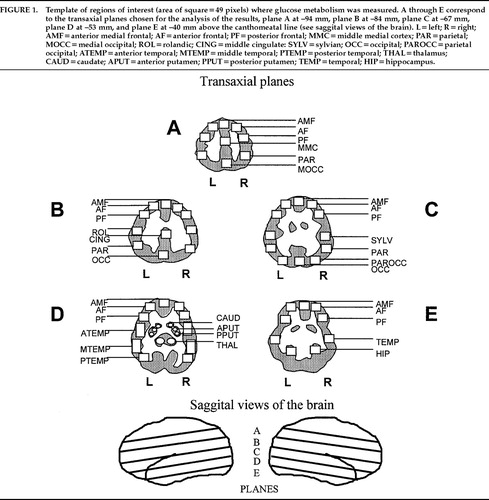
FIGURE 1. Template of regions of interest (area of square=49 pixels) where glucose metabolism was measured. A through E correspond to the transaxial planes chosen for the analysis of the results, plane A at –94 mm, plane B at –84 mm, plane C at –67 mm, plane D at –53 mm, and plane E at –40 mm above the canthomeatal line (see saggital views of the brain). L=left; R=right; AMF=anterior medial frontal; AF=anterior frontal; PF=posterior frontal; MMC=middle medial cortex; PAR=parietal; MOCC=medial occipital; ROL=rolandic; CING=middle cingulate: SYLV=sylvian; OCC=occipital; PAROCC=parietal occipital; ATEMP=anterior temporal; MTEMP=middle temporal; PTEMP=posterior temporal; THAL=thalamus; CAUD=caudate; APUT=anterior putamen; PPUT=posterior putamen; TEMP=temporal; HIP=hippocampus.
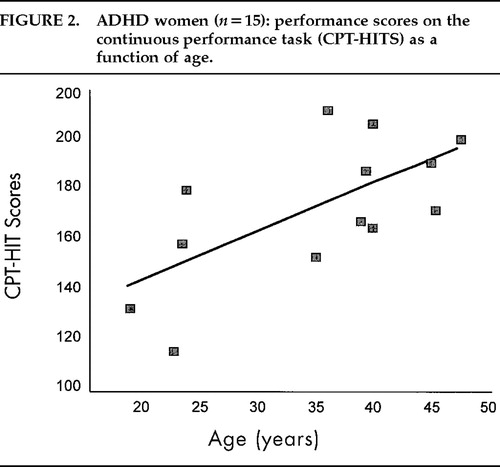
FIGURE 2. ADHD women (n=15): performance scores on the continuous performance task (CPT-HITS) as a function of age.
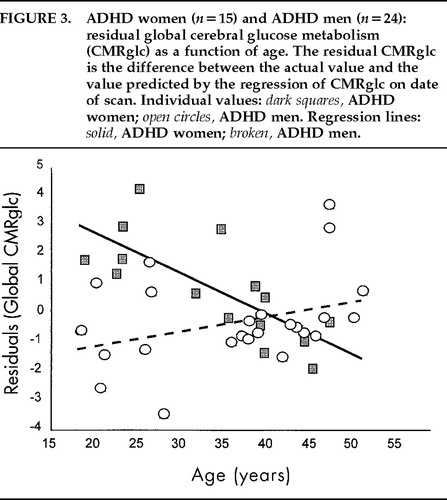
FIGURE 3. ADHD women (n=15) and ADHD men (n=24): residual global cerebral glucose metabolism (CMRglc) as a function of age. The residual CMRglc is the difference between the actual value and the value predicted by the regression of CMRglc on date of scan.
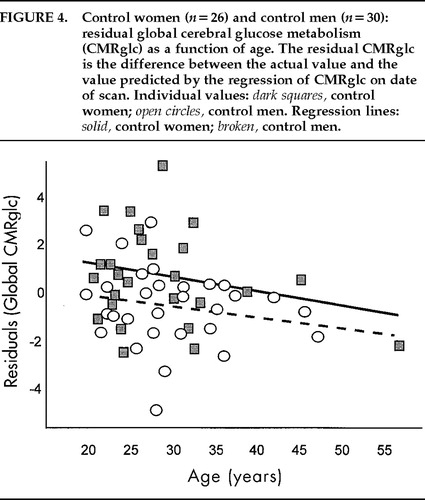
FIGURE 4. Control women (n=26) and control men (n=30): residual global cerebral glucose metabolism (CMRglc) as a function of age. The residual CMRglc is the difference between the actual value and the value predicted by the regression of CMRglc on date of scan. Individual values: dark squares, control women; open circles, control men. Regression lines: solid, control women; broken, control men.
![]() |
![]() |
![]() |
![]() |
![]() |
1. Andreason PJ, Zametkin AJ, Guo AC, et al: Gender-related differences in regional cerebral glucose metabolism in normal volunteers. Psychiatry Res 1994; 51:175–183Crossref, Medline, Google Scholar
2. Zametkin A, Liebenauer L, Fitzgerald G, et al: Brain metabolism in teenagers with attention deficit hyperactivity disorder. Arch Gen Psychiatry 1993; 50:333–340Crossref, Medline, Google Scholar
3. Zametkin AJ, Nordahl TE, Gross M, et al: Cerebral glucose metabolism in adults with hyperactivity of childhood onset. N Engl J Med 1990; 323:1361–1366Crossref, Medline, Google Scholar
4. Ernst M, Liebenauer LL, King AC, et al: Reduced brain metabolism in hyperactive girls. J Am Acad Child Adolesc Psychiatry 1994; 33:858–868Crossref, Medline, Google Scholar
5. Cohen RM, Semple WE, Gross M, et al: Metabolic brain pattern of sustained auditory discrimination. Exp Brain Res 1992; 92:165–172Crossref, Medline, Google Scholar
6. Goyer PF, Andreason PJ, Semple WE, et al: Positron-emission tomography and personality disorders. Neuropsychopharmacology 1994; 10:21–28Crossref, Medline, Google Scholar
7. Cohen RM, Nordahl TE, Semple WE, et al: The brain metabolic patterns of clozapine and fluphenazine-treated patients with schizophrenia during a continuous performance task. Arch Gen Psychiatry 1997; 54:481–486Crossref, Medline, Google Scholar
8. Endicott J, Spitzer RL: A diagnostic interview: the Schedule for Affective Disorders and Schizophrenia. Arch Gen Psychiatry 1978; 35:837–844Crossref, Medline, Google Scholar
9. Wender PH, Reimherr FW, Wood DR: Attention deficit disorder (“minimal brain dysfunction”) in adults: a replication study of diagnosis and drug treatment. Arch Gen Psychiatry 1981; 38:449–456Crossref, Medline, Google Scholar
10. Conners CK: A teacher rating scale for use in drug studies with children. Am J Psychiatry 1969; 126:884–888Crossref, Medline, Google Scholar
11. Cohen RM, Semple WE, Gross M, et al: Functional localization of sustained attention: comparison to sensory stimulation in the absence of instruction. Neuropsychiatry Neuropsychol Behav Neurol 1988; 1:3–20Google Scholar
12. Spielberger CD, Gorsuch RL, Lushene RE: Manual for the State-Trait Anxiety Inventory. Palo Alto, CA, Consulting Psychologist Press, 1970Google Scholar
13. Gur RC, Gur RE, Resnick SM, et al: The effect of anxiety on cortical cerebral blood flow and metabolism. J Cereb Blood Flow Metab 1987; 7:173–177Crossref, Medline, Google Scholar
14. Giordani B, Boivin MJ, Berent S, et al: Anxiety and cerebral cortical metabolism in normal persons. Psychiatry Research Neuroimaging 1990; 35:49–60Crossref, Medline, Google Scholar
15. Carson RE, Daube-Witherspoon ME, Green MV: A method for post-injection PET transmission measurements with a rotating source. J Nucl Med 1988; 29:1558–1567Medline, Google Scholar
16. Brooks RA: Alternative formula for glucose utilization using labeled deoxyglucose. J Nucl Med 1982; 23:538–539Medline, Google Scholar
17. Huang S-C, Phelps ME, Hoffman EJ, et al: Noninvasive determination of local cerebral metabolic rate of glucose in man. Am J Physiol 1980; 238:E69–E82Google Scholar
18. Matsui T, Hirano A: An Atlas of the Human Brain for Computerized Tomography. New York, Igaku-Shoin, 1978Google Scholar
19. Barkley R: Developmental course and adult outcome, in Attention Deficit Hyperactivity Disorder: A Handbook for Diagnosis and Treatment, edited by Barkley R. New York, Guilford, 1990, pp 114–129Google Scholar
20. Haier RJ, Siegel BV Jr, MacLachlan A, et al: Regional glucose metabolic changes after learning a complex visuospatial/motor task: a positron emission tomographic study. Brain Res 1992; 570:134–143Crossref, Medline, Google Scholar
21. Esposito G, Van Horn JD, Weinberger DR, et al: Gender differences in cerebral blood flow as a function of cognitive state with PET. J Nucl Med 1996; 37:559–564Medline, Google Scholar
22. Devous MD, Stokely EM, Chehabi HH, et al: Normal distribution of regional blood flow measured by dynamic single-photon emission tomography. J Cereb Blood Flow Metab 1986; 6:95–104Crossref, Medline, Google Scholar
23. Shaw TG, Mortel KF, Meyer JS, et al: Cerebral blood flow changes in benign aging and cerebrovascular disease. Neurology 1984; 34:855–862Crossref, Medline, Google Scholar
24. Davis SM, Ackerman RH, Correia JA, et al: Cerebral blood flow and cerebrovascular CO2 reactivity in stroke-age normal controls. Neurology 1983; 33:391–399Crossref, Medline, Google Scholar
25. American Psychiatric Association: Diagnostic and Statistical Manual of Mental Disorders, 4th edition. Washington, DC, American Psychiatric Association, 1994Google Scholar
26. Ackerman P, Dykman RA, Oglesby D: Sex and group differences in reading and attention disordered children with and without hyperkinesis. Journal of Learning Disabilities 1983; 16:407–414Crossref, Medline, Google Scholar
27. Berry C, Shaywitz S, Shaywitz B: Girls with attention deficit disorder: a silent minority? A report on behavioral and cognitive characteristics. Pediatrics 1985; 76:801–809Medline, Google Scholar
28. Barkley R: Hyperactive girls and boys: stimulant drug effects on mother-child interactions. J Child Psychol Psychiatry 1989; 30:379–390Crossref, Medline, Google Scholar
29. Brown R, Madan-Swain A, Baldwin K: Gender differences in a clinic-referred sample of attention deficit-disordered children. Child Psychiatry Hum Dev 1991; 22:111–128Crossref, Medline, Google Scholar
30. James A, Taylor E: Sex differences in the hyperkinetic syndrome of childhood. J Child Psychol Psychiatry 1990; 31:437–446Crossref, Medline, Google Scholar
31. Shaywitz SE, Shaywitz BA: Attention deficit disorder: current perspectives. Pediatr Neurol 1987; 3:129–135Crossref, Medline, Google Scholar
32. Barkley RA, Anastastopoulos AD, Guevremont DC, et al: Adolescents with ADHD: patterns of behavioral adjustment, academic functioning, and treatment utilization. J Am Acad Child Adolesc Psychiatry 1990; 30:752–761Google Scholar
33. Befera M, Barkley R: Hyperactive and normal girls and boys: mother-child interaction, parent psychiatric status and child psychopathology. J Child Psychol Psychiatry 1985; 26:439–452Crossref, Medline, Google Scholar
34. Breen M, Barkley R: Child psychopathy and parenting stress in girls and boys having attention deficit disorder with hyperactivity. Journal of Pediatric Psychology 1988; 13:265–280Crossref, Medline, Google Scholar
35. Breen M: Cognitive and behavioral differences in ADHD boys and girls. J Child Psychol Psychiatry 1989; 30:711–716Crossref, Medline, Google Scholar
36. de Haas PA, Young RD: Attention styles of hyperactive and normal girls. Journal of Abnormal Child Psychology 1984; 12:531–546Crossref, Medline, Google Scholar
37. de Haas PA: Attention styles and peer relationships of hyperactive and normal girls and boys. Journal of Abnormal Child Psychology 1986; 14:457–467Crossref, Medline, Google Scholar
38. Hampson E, Kimura D: Reciprocal effects of hormonal fluctuations on human motor and perceptual-spatial skills. Behav Neurosci 1988; 2:456–459Crossref, Google Scholar
39. Baxter LR, Mazziotta JC, Phelps ME, et al: Cerebral glucose metabolic rates in normal human females versus normal males. Psychiatry Res 1987; 21:237–245Crossref, Medline, Google Scholar
40. Yoshii F, Barker WW, Chang JY, et al: Sensitivity of cerebral glucose metabolism to age, gender, brain volume, brain atrophy, and cerebrovascular risk factors. J Cereb Blood Flow Metab 1988; 8:654–661Crossref, Medline, Google Scholar
41. Miura SA, Schapiro MB, Grady CL, et al: Effect of gender on glucose utilization rates in healthy humans: a positron emission tomography study. J Neurosci Res 1990; 27:500–504Crossref, Medline, Google Scholar
42. Gur RC, Mozley LH, Mozley PD, et al: Sex differences in regional cerebral glucose metabolism during a resting state. Science 1995; 267:528–531Crossref, Medline, Google Scholar
43. Ernst M, Zametkin AJ: The interface of neurochemistry, genetics, and neuroimaging in attention-deficit hyperactivity disorder, in Psychopharmacology: The Fourth Generation of Progress, edited by Bloom FE, Kupfer DJ. New York, Raven, 1995, pp 1643–1651Google Scholar
44. Weiss G, Hechtman LT: Hyperactive Children Grown Up. New York, Guilford, 1986Google Scholar
45. Mannuzza S, Klein RG, Bonagura N, et al: Hyperactive boys almost grown up, V: replication of psychiatric status. Arch Gen Psychiatry 1991; 48:77–83Crossref, Medline, Google Scholar
46. Mannuzza S, Klein RG, Bessler A, et al: Adult outcome of hyperactive boys: educational achievement, occupational rank, and psychiatric status. Arch Gen Psychiatry 1993; 50:565–576Crossref, Medline, Google Scholar
47. Kuhl DE, Metter EJ, Riege WH, et al: Effects of human aging on patterns of local cerebral glucose utilization determined by the [18F] fluorodeoxyglucose method. J Cereb Blood Flow Metab 1982; 2:163–171Crossref, Medline, Google Scholar
48. Hawkins RA, Mazziotta JC, Phelps ME, et al: Cerebral glucose metabolism as a function of age in man: influence of the rate constants in the fluorodeoxyglucose method. J Cereb Blood Flow Metab 1983; 3:250–253Crossref, Medline, Google Scholar
49. de Leon MJ, George AE, Ferris SH, et al: Positron emission tomography and computed tomography assessments of the aging human brain. J Comput Assist Tomogr 1984; 8:88–94Crossref, Medline, Google Scholar
50. Duara R, Grady C, Haxby J, et al: Human brain glucose utilization and cognitive function in relation to age. Ann Neurol 1984; 16:702–713Crossref, Google Scholar
51. Duara R, Grady C, Haxby J, et al: Positron emission tomography in Alzheimer's disease. Neurology 1986; 36:879–887Crossref, Medline, Google Scholar
52. de Leon MJ, George AE, Tomanelli J, et al: Positron emission tomography studies of normal aging: a replication of PET III and 18-FDG using PET VI and 11-CDG. Neurobiol Aging 1987; 8:319–323Crossref, Medline, Google Scholar
53. Loessner A, Alavi A, Lewandrowski KU, et al: Regional cerebral function determined by FDG-PET in healthy volunteers: normal patterns and changes with age. J Nucl Med 1995; 36:1141–1149Medline, Google Scholar
54. Frackowiak RSJ, Lenzi G-L, Jones T, et al: Quantitative measurement of regional cerebral blood flow and oxygen metabolism in man using 15O and positron emission tomography: theory, procedure, and normal values. J Comput Assist Tomogr 1980; 4:727–736Crossref, Medline, Google Scholar
55. Lenzi GL, Frackowiak RSJ, Jones T, et al: CMRO2 and CBF by the oxygen-15 inhalation technique. Eur Neurol 1981; 20:285–290Crossref, Medline, Google Scholar
56. Pantano P, Baron JC, Grandie PL, et al: Regional cerebral blood flow and oxygen consumption in human aging. Stroke 1984; 15:635–641Crossref, Medline, Google Scholar
57. Yamaguchi T, Kanno I, Uemura K, et al: Reduction in regional cerebral metabolic rate of oxygen during human aging. Stroke 1986; 17:1220–1228Crossref, Medline, Google Scholar
58. Itoh M, Hatazawa J, Mijazawa H, et al: Stability of cerebral blood flow and oxygen metabolism during normal aging. Gerontology 1990; 36:43–48Crossref, Medline, Google Scholar
59. Martin AJ, Friston KJ, Colebatch JG, et al: Decreases in regional cerebral blood flow with normal aging. J Cereb Blood Flow Metab 1991; 11:684–689Crossref, Medline, Google Scholar
60. Takada H, Nagata K, Hirata Y, et al: Age-related decline of cerebral oxygen metabolism in normal population detected with positron emission tomography. Neurol Res 1992; 14:128–131Crossref, Medline, Google Scholar
61. Naritomi H, Meyer JS, Sakai F: Effects of advancing age on regional cerebral blood flow. Arch Neurol 1979; 36:410–416Crossref, Medline, Google Scholar
62. Melamed E, Lavy S, Bentin S, et al: Reduction in regional cerebral blood flow during normal aging in man. Stroke 1980; 11:31–35Crossref, Medline, Google Scholar
63. Amano T, Meyer JS, Okabe T, et al: Stable Xenon CT cerebral blood flow measurements computed by a single compartment-double integration model in normal aging and dementia. J Comput Assist Tomogr 1982; 6:923–932Crossref, Medline, Google Scholar
64. Globus M, Cooper G, Melamed E: Reduction in regional cerebral blood flow during normal aging is not limited to elderly subjects. Monogr Neural Sci 1984; 11:139–143Medline, Google Scholar
65. Gur RC, Gur RE, Obrist WD, et al: Age and regional cerebral blood flow at rest and during cognitive activity. Arch Gen Psychiatry 1987; 44:617–621Crossref, Medline, Google Scholar
66. Imai A, Meyer JS, Kobari M, et al: LCBF values decline while L values increase during normal human aging measured by stable xenon-enhanced computed tomography. Neuroradiology 1988; 30:463–472Crossref, Medline, Google Scholar
67. Takeda S, Matsuzawa T, Matsui H: Age-related changes in regional cerebral blood flow and brain volume in healthy subjects. J Am Geriatr Soc 1988; 36:293–297Crossref, Medline, Google Scholar
68. Hagstadius S, Risberg J: Regional cerebral blood flow characteristics and variations with age in resting normal subjects. Brain Cogn 1989; 10:28–43Crossref, Medline, Google Scholar
69. Waldemar G, Hasselbalch SG, Andersen AR, et al: 99mTc-d,1-HMPAO and SPECT of the brain in normal aging. J Cereb Blood Flow Metab 1991; 11:508–521Crossref, Medline, Google Scholar
70. Dastur DK, Lane MH, Hansen DB, et al: Effects of aging on cerebral circulation and metabolism in man, in Human Aging: A Biological and Behavioral Study, edited by Birren JE, Butler RN, Greenhouse SW, et al. Bethesda, MD, National Institute of Mental Health, 1963, pp 59–76Google Scholar